Abstract
Depolarization of neurons promotes Ca2+ influx through voltage-activated channels, raising the intracellular Ca2+ concentration ([Ca2+]i). The consequences of such changes in [Ca2+]i for mitochondrial function were assessed in single, freshly dissociated mammalian neurons. Microfluorimetric techniques were used to measure [Ca2+]i, mitochondrial membrane potential [delta psi m, Rhodamine 123 (Rh 123) fluorescence], NAD(P)H/NAD(P)+ autofluorescence and flavoprotein autofluorescence combined with whole-cell voltage-clamp techniques. Brief (100-500 ms) depolarization of the cell membrane by high K+ or by voltage commands raised [Ca2+]i and depolarized delta psi m. The change in delta psi m was dependent on extracellular Ca2+. Under voltage-clamp control of the cell membrane, the voltage-dependence of the change in Rh 123 fluorescence reflected that of the Ca2+ current. The response was reduced by Ca2+ buffers introduced into the cell. The behaviour of this signal is thus consistent with a mitochondrial response to raised [Ca2+]i and does not reflect the change in cell membrane potential per se. Similar stimuli caused a rapid decrease of NAD(P)H autofluorescence, followed by an increase which could last several minutes. Flavoprotein fluorescence increased transiently, followed by a decrease lasting for several minutes. These signals indicate an initial oxidation of NAD(P)H and FADH, followed by a prolonged increase in the reduced state of both coenzymes. All these changes were dependent on extracellular [Ca2+]. Raising [Ca2+]i again during the period of NAD+ reduction caused an oxidizing response. Ruthenium Red applied to the cells (i) reduced both the Ca2+ current and the depolarization-induced [Ca2+]i transient and (ii) directly quenched Rh 123 fluorescence. When introduced into the cells with patch pipettes, it prevented the changes in autofluorescence without interfering with the Ca2+ conductance. Oligomycin blocked neither the response of delta psi m nor of NADH autofluorescence, suggesting that the signals do not reflect a response to falling ATP/ADP.Pi ratios as a consequence of the high [Ca2+]i. The changes in NADH autofluorescence were sustained in the presence of iodoacetic acid with pyruvate as substrate. Thus brief physiological elevations of [Ca2+]i depolarize delta psi m, probably through Ca2+ cycling across the mitochondrial inner membrane. The changes in autofluorescence are consistent with (i) increased respiration which could result from the depolarization of delta psi m, followed rapidly by (ii) increased activity of the Ca(2+)-dependent intramitochondrial enzymes. Changes in [Ca2+]i within a physiological range may thus promote significant and long-lasting changes in mitochondrial energy production.
Full text
PDF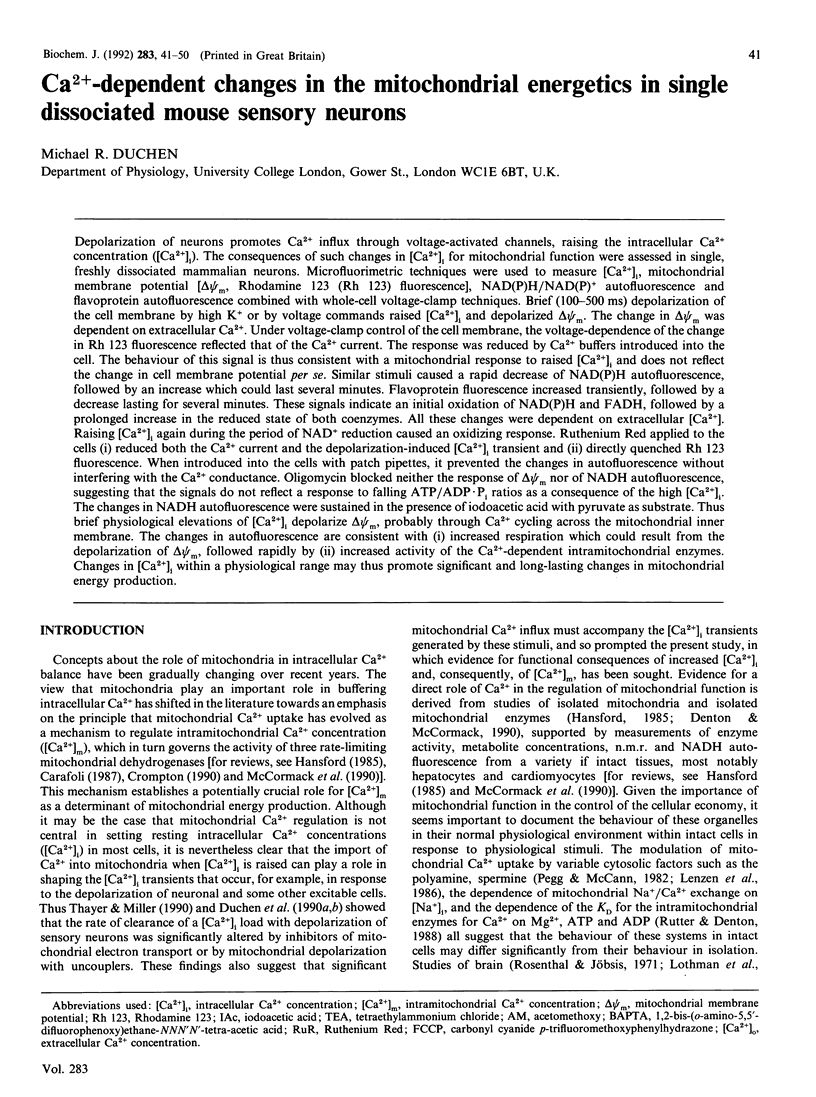
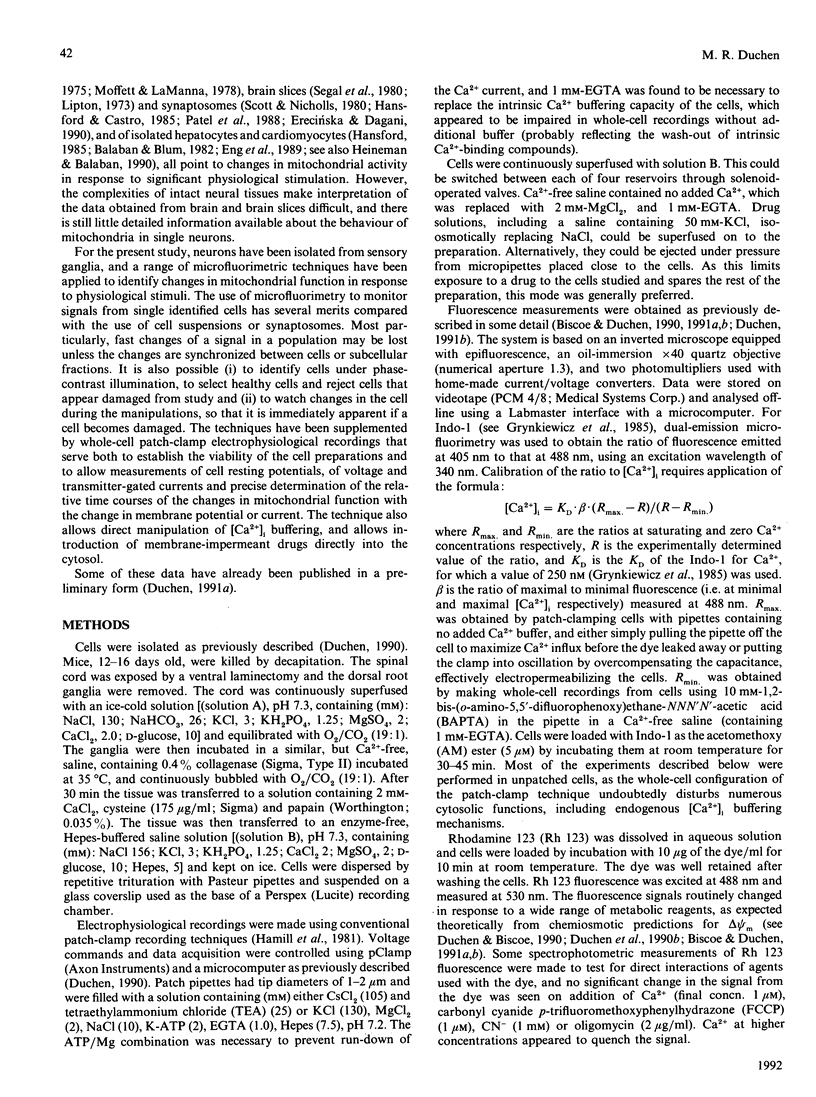
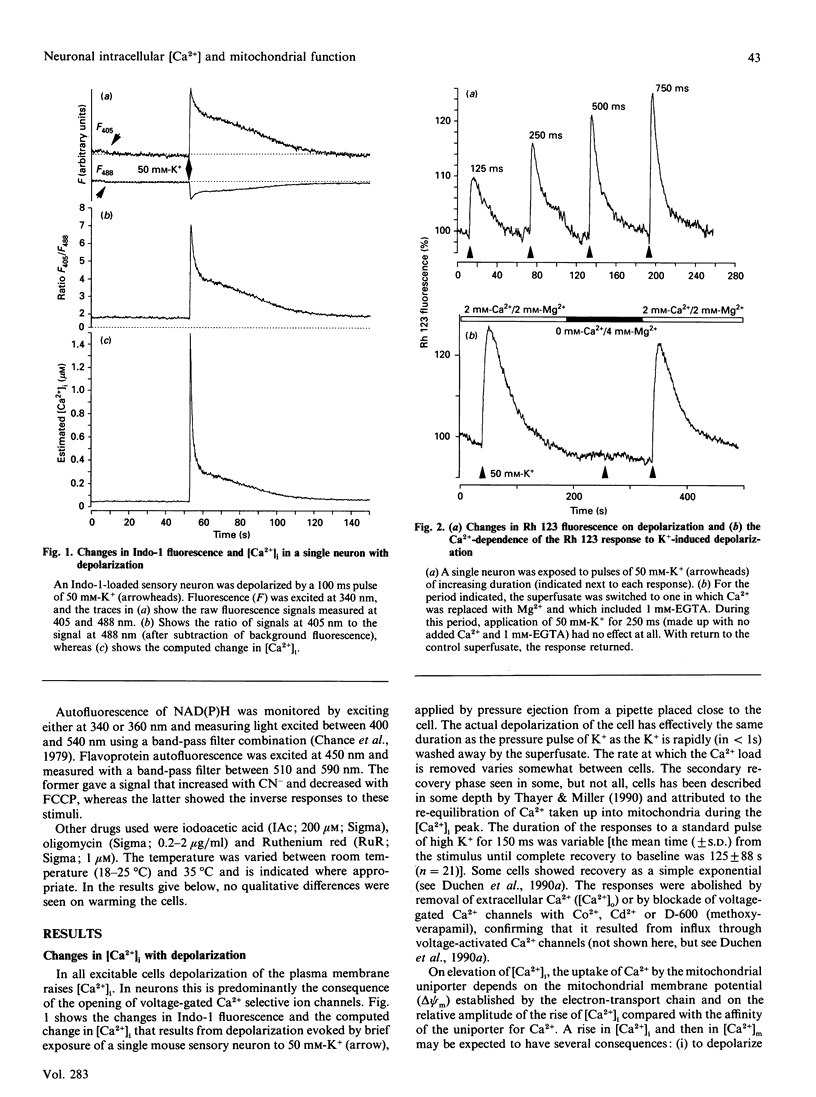
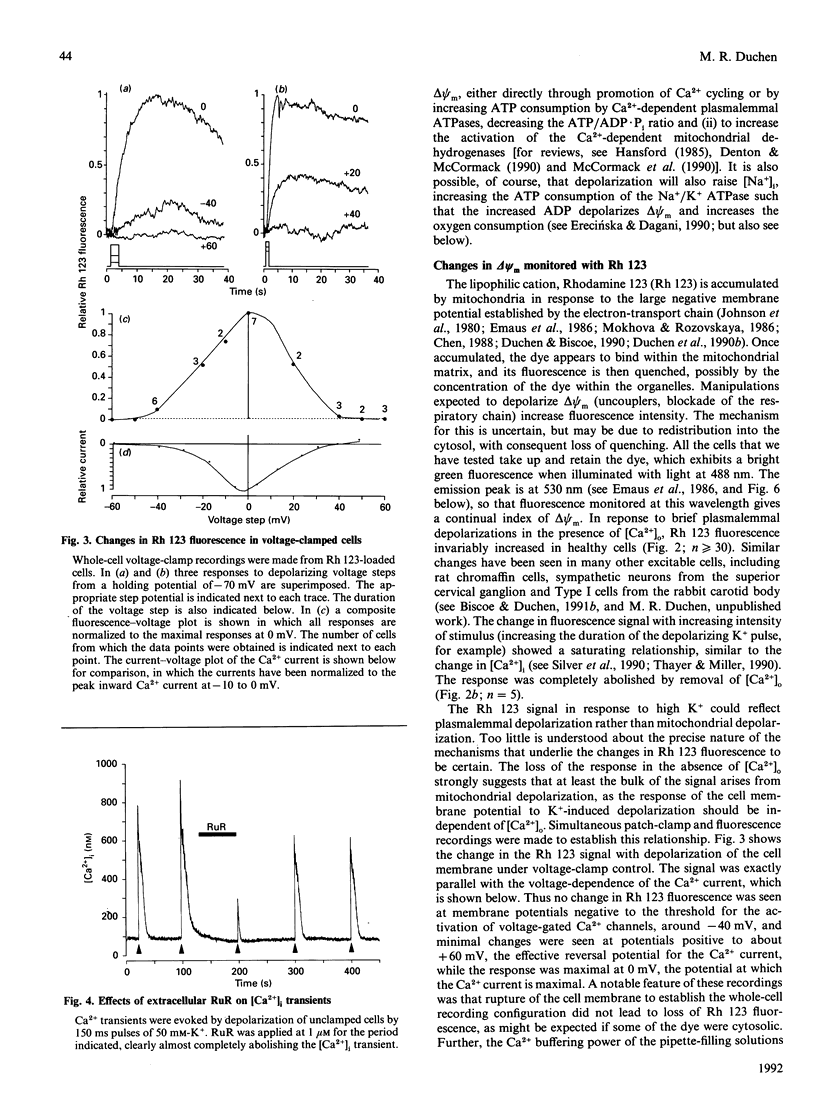
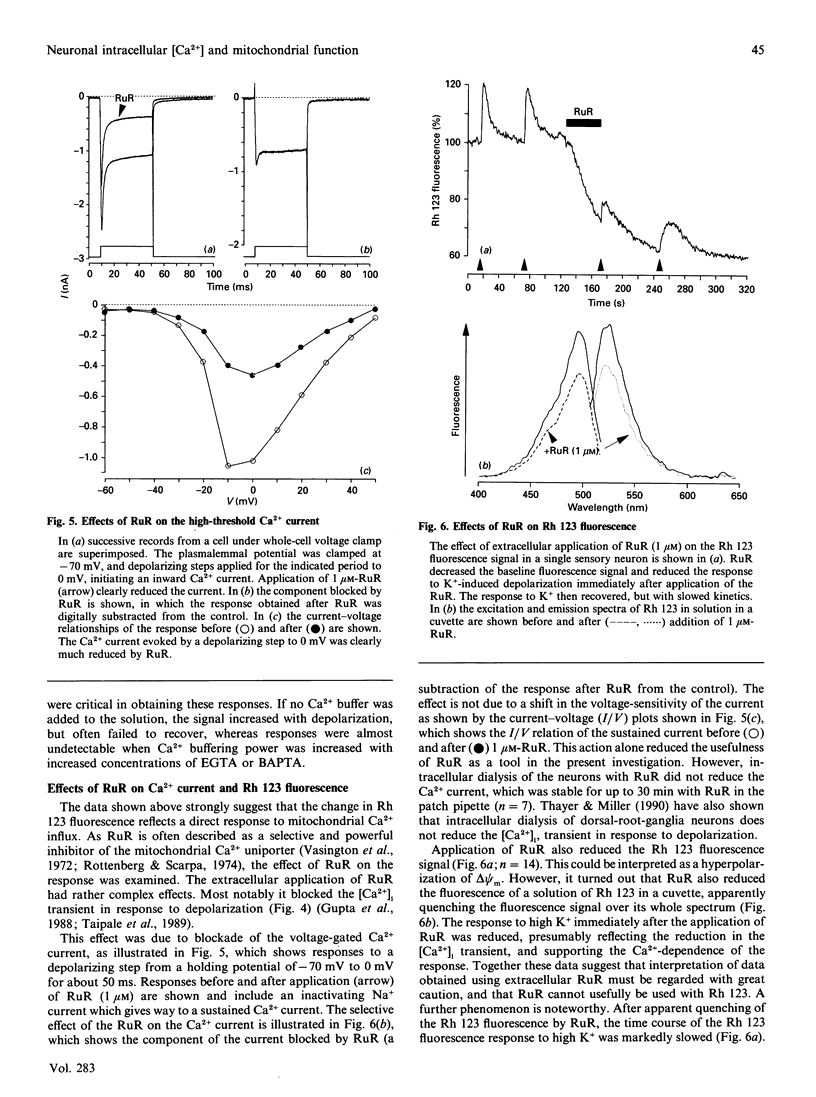
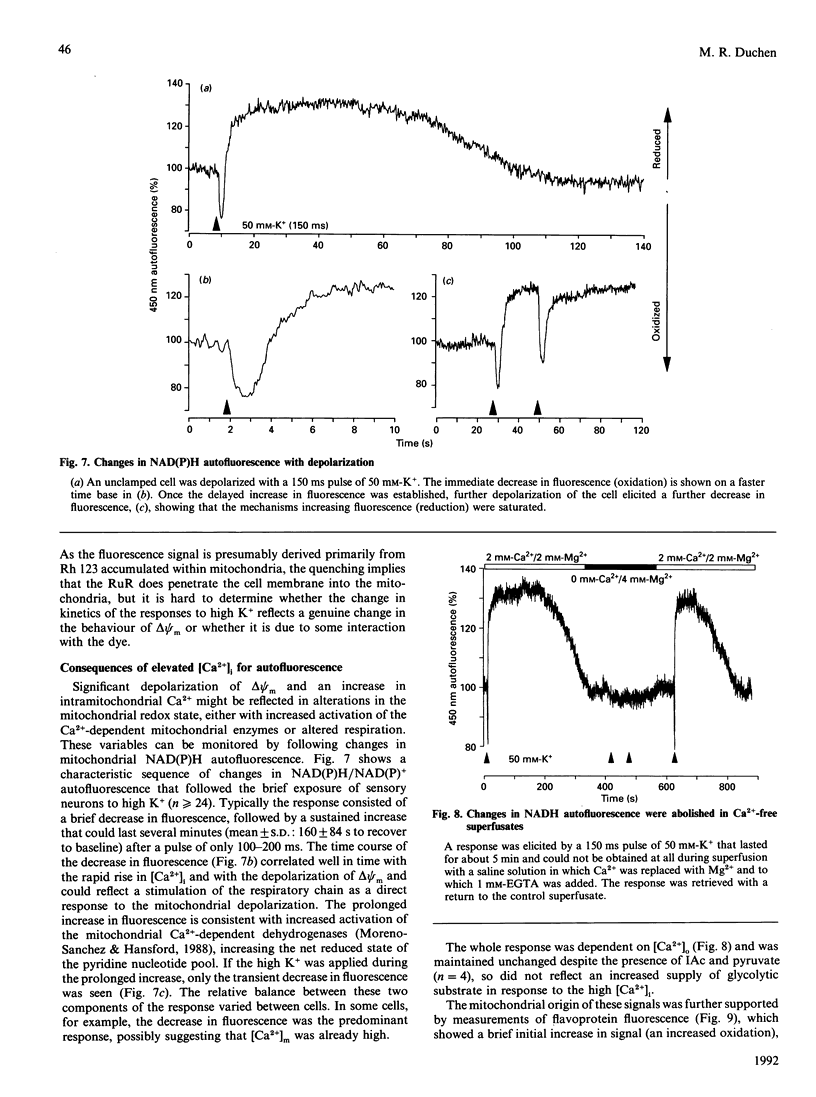
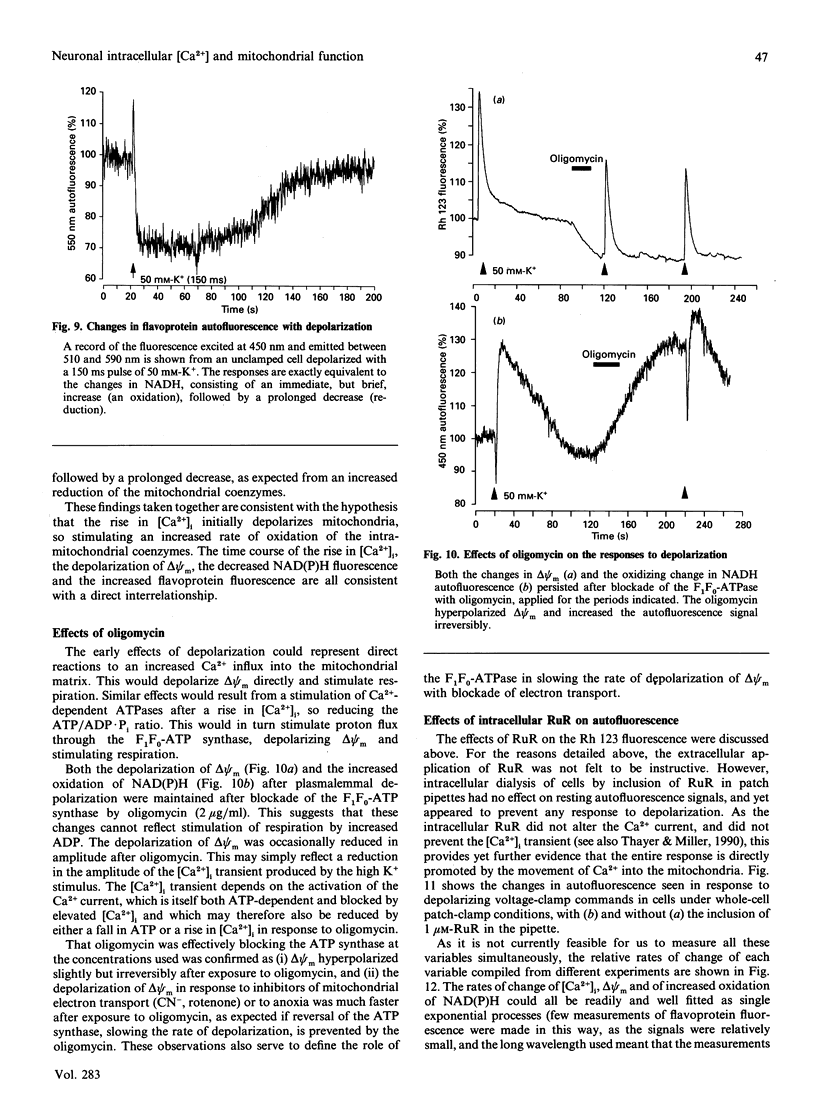
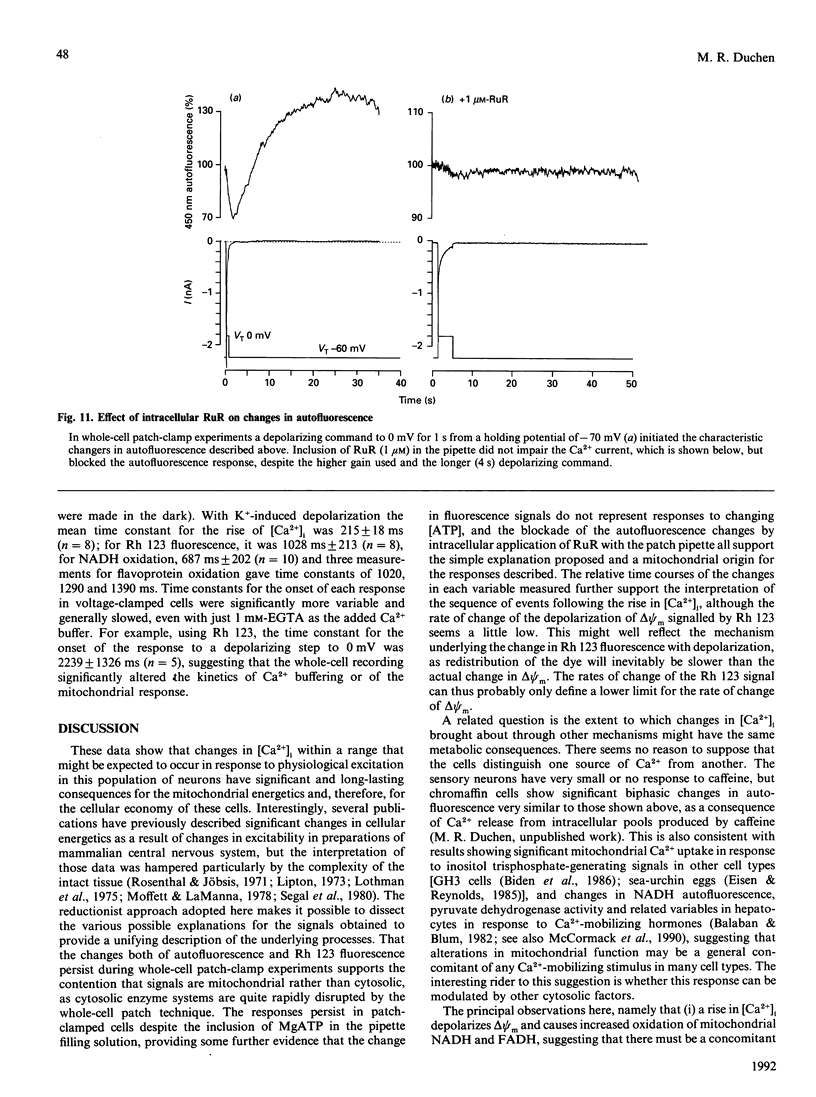
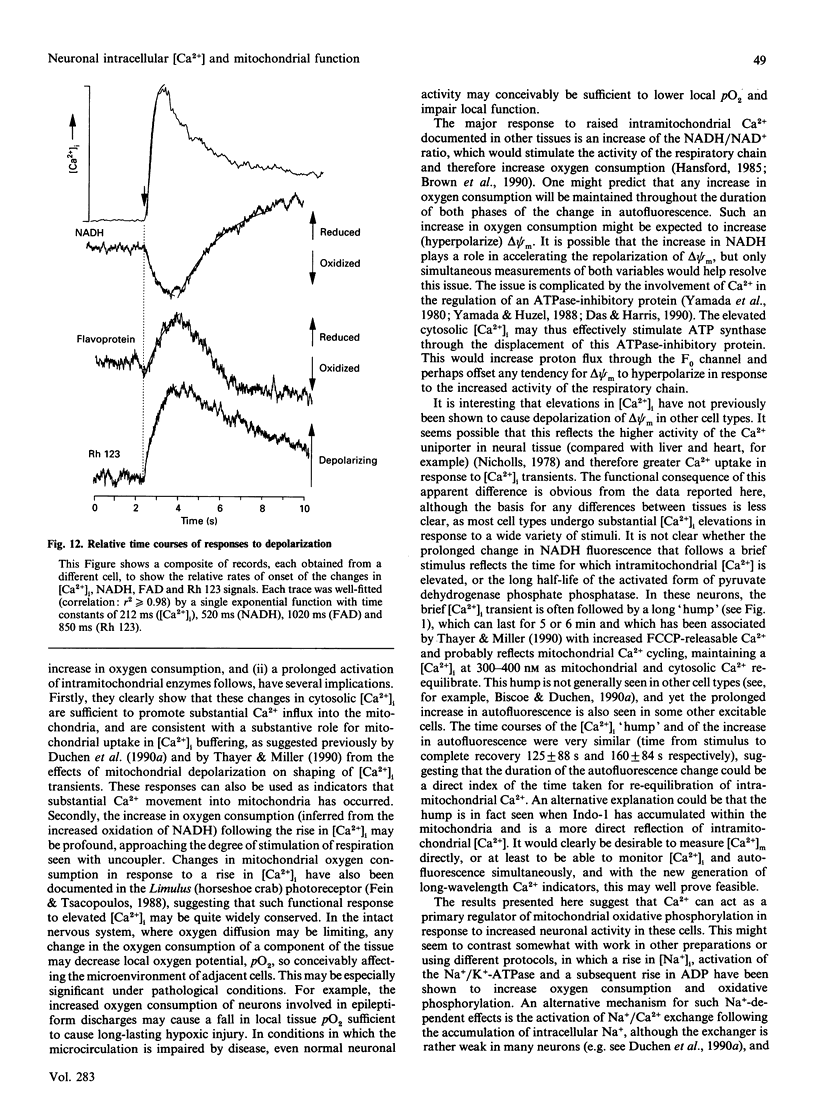
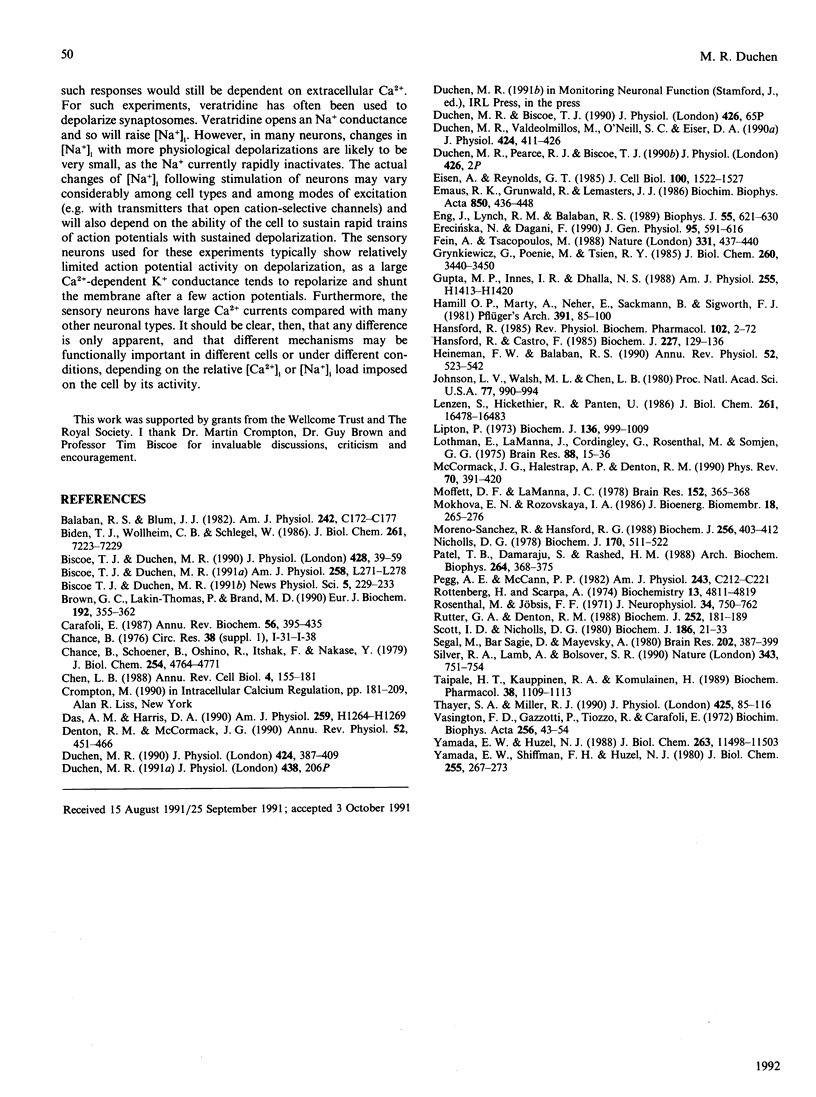
Selected References
These references are in PubMed. This may not be the complete list of references from this article.
- Balaban R. S., Blum J. J. Hormone-induced changes in NADH fluorescence and O2 consumption of rat hepatocytes. Am J Physiol. 1982 Mar;242(3):C172–C177. doi: 10.1152/ajpcell.1982.242.3.C172. [DOI] [PubMed] [Google Scholar]
- Biden T. J., Wollheim C. B., Schlegel W. Inositol 1,4,5-trisphosphate and intracellular Ca2+ homeostasis in clonal pituitary cells (GH3). Translocation of Ca2+ into mitochondria from a functionally discrete portion of the nonmitochondrial store. J Biol Chem. 1986 Jun 5;261(16):7223–7229. [PubMed] [Google Scholar]
- Biscoe T. J., Duchen M. R. Responses of type I cells dissociated from the rabbit carotid body to hypoxia. J Physiol. 1990 Sep;428:39–59. doi: 10.1113/jphysiol.1990.sp018199. [DOI] [PMC free article] [PubMed] [Google Scholar]
- Brown G. C., Lakin-Thomas P. L., Brand M. D. Control of respiration and oxidative phosphorylation in isolated rat liver cells. Eur J Biochem. 1990 Sep 11;192(2):355–362. doi: 10.1111/j.1432-1033.1990.tb19234.x. [DOI] [PubMed] [Google Scholar]
- Carafoli E. Intracellular calcium homeostasis. Annu Rev Biochem. 1987;56:395–433. doi: 10.1146/annurev.bi.56.070187.002143. [DOI] [PubMed] [Google Scholar]
- Chance B., Schoener B., Oshino R., Itshak F., Nakase Y. Oxidation-reduction ratio studies of mitochondria in freeze-trapped samples. NADH and flavoprotein fluorescence signals. J Biol Chem. 1979 Jun 10;254(11):4764–4771. [PubMed] [Google Scholar]
- Chen L. B. Mitochondrial membrane potential in living cells. Annu Rev Cell Biol. 1988;4:155–181. doi: 10.1146/annurev.cb.04.110188.001103. [DOI] [PubMed] [Google Scholar]
- Das A. M., Harris D. A. Defects in regulation of mitochondrial ATP synthase in cardiomyocytes from spontaneously hypertensive rats. Am J Physiol. 1990 Oct;259(4 Pt 2):H1264–H1269. doi: 10.1152/ajpheart.1990.259.4.H1264. [DOI] [PubMed] [Google Scholar]
- Denton R. M., McCormack J. G. Ca2+ as a second messenger within mitochondria of the heart and other tissues. Annu Rev Physiol. 1990;52:451–466. doi: 10.1146/annurev.ph.52.030190.002315. [DOI] [PubMed] [Google Scholar]
- Duchen M. R. Effects of metabolic inhibition on the membrane properties of isolated mouse primary sensory neurones. J Physiol. 1990 May;424:387–409. doi: 10.1113/jphysiol.1990.sp018073. [DOI] [PMC free article] [PubMed] [Google Scholar]
- Duchen M. R., Valdeolmillos M., O'Neill S. C., Eisner D. A. Effects of metabolic blockade on the regulation of intracellular calcium in dissociated mouse sensory neurones. J Physiol. 1990 May;424:411–426. doi: 10.1113/jphysiol.1990.sp018074. [DOI] [PMC free article] [PubMed] [Google Scholar]
- Eisen A., Reynolds G. T. Source and sinks for the calcium released during fertilization of single sea urchin eggs. J Cell Biol. 1985 May;100(5):1522–1527. doi: 10.1083/jcb.100.5.1522. [DOI] [PMC free article] [PubMed] [Google Scholar]
- Emaus R. K., Grunwald R., Lemasters J. J. Rhodamine 123 as a probe of transmembrane potential in isolated rat-liver mitochondria: spectral and metabolic properties. Biochim Biophys Acta. 1986 Jul 23;850(3):436–448. doi: 10.1016/0005-2728(86)90112-x. [DOI] [PubMed] [Google Scholar]
- Eng J., Lynch R. M., Balaban R. S. Nicotinamide adenine dinucleotide fluorescence spectroscopy and imaging of isolated cardiac myocytes. Biophys J. 1989 Apr;55(4):621–630. doi: 10.1016/S0006-3495(89)82859-0. [DOI] [PMC free article] [PubMed] [Google Scholar]
- Erecińska M., Dagani F. Relationships between the neuronal sodium/potassium pump and energy metabolism. Effects of K+, Na+, and adenosine triphosphate in isolated brain synaptosomes. J Gen Physiol. 1990 Apr;95(4):591–616. doi: 10.1085/jgp.95.4.591. [DOI] [PMC free article] [PubMed] [Google Scholar]
- Fein A., Tsacopoulos M. Activation of mitochondrial oxidative metabolism by calcium ions in Limulus ventral photoreceptor. Nature. 1988 Feb 4;331(6155):437–440. doi: 10.1038/331437a0. [DOI] [PubMed] [Google Scholar]
- Grynkiewicz G., Poenie M., Tsien R. Y. A new generation of Ca2+ indicators with greatly improved fluorescence properties. J Biol Chem. 1985 Mar 25;260(6):3440–3450. [PubMed] [Google Scholar]
- Hamill O. P., Marty A., Neher E., Sakmann B., Sigworth F. J. Improved patch-clamp techniques for high-resolution current recording from cells and cell-free membrane patches. Pflugers Arch. 1981 Aug;391(2):85–100. doi: 10.1007/BF00656997. [DOI] [PubMed] [Google Scholar]
- Hansford R. G., Castro F. Role of Ca2+ in pyruvate dehydrogenase interconversion in brain mitochondria and synaptosomes. Biochem J. 1985 Apr 1;227(1):129–136. doi: 10.1042/bj2270129. [DOI] [PMC free article] [PubMed] [Google Scholar]
- Hansford R. G. Relation between mitochondrial calcium transport and control of energy metabolism. Rev Physiol Biochem Pharmacol. 1985;102:1–72. doi: 10.1007/BFb0034084. [DOI] [PubMed] [Google Scholar]
- Heineman F. W., Balaban R. S. Control of mitochondrial respiration in the heart in vivo. Annu Rev Physiol. 1990;52:523–542. doi: 10.1146/annurev.ph.52.030190.002515. [DOI] [PubMed] [Google Scholar]
- Johnson L. V., Walsh M. L., Chen L. B. Localization of mitochondria in living cells with rhodamine 123. Proc Natl Acad Sci U S A. 1980 Feb;77(2):990–994. doi: 10.1073/pnas.77.2.990. [DOI] [PMC free article] [PubMed] [Google Scholar]
- Lenzen S., Hickethier R., Panten U. Interactions between spermine and Mg2+ on mitochondrial Ca2+ transport. J Biol Chem. 1986 Dec 15;261(35):16478–16483. [PubMed] [Google Scholar]
- Lipton P. Effects of membrane depolarization on nicotinamide nucleotide fluorescence in brain slices. Biochem J. 1973 Dec;136(4):999–1009. doi: 10.1042/bj1360999. [DOI] [PMC free article] [PubMed] [Google Scholar]
- Lothman E., Lamanna J., Cordingley G., Rosenthal M., Somjen G. Responses of electrical potential, potassium levels, and oxidative metabolic activity of the cerebral neocortex of cats. Brain Res. 1975 Apr 25;88(1):15–36. doi: 10.1016/0006-8993(75)90943-9. [DOI] [PubMed] [Google Scholar]
- McCormack J. G., Halestrap A. P., Denton R. M. Role of calcium ions in regulation of mammalian intramitochondrial metabolism. Physiol Rev. 1990 Apr;70(2):391–425. doi: 10.1152/physrev.1990.70.2.391. [DOI] [PubMed] [Google Scholar]
- Moffett D. F., LaManna J. C. Contributions of glycolysis and oxidative metabolism to recovery from electrical pulses in the isolated toad brain. Brain Res. 1978 Aug 25;152(2):365–368. doi: 10.1016/0006-8993(78)90265-2. [DOI] [PubMed] [Google Scholar]
- Mokhova E. N., Rozovskaya I. A. The effects of mitochondrial energetics inhibitors on the fluorescence of potential-sensitive dyes rhodamine 123 and diS-C3-(5) in lymphocyte suspensions. J Bioenerg Biomembr. 1986 Aug;18(4):265–276. doi: 10.1007/BF00743047. [DOI] [PubMed] [Google Scholar]
- Moreno-Sánchez R., Hansford R. G. Dependence of cardiac mitochondrial pyruvate dehydrogenase activity on intramitochondrial free Ca2+ concentration. Biochem J. 1988 Dec 1;256(2):403–412. doi: 10.1042/bj2560403. [DOI] [PMC free article] [PubMed] [Google Scholar]
- Nicholls D. G. Calcium transport and porton electrochemical potential gradient in mitochondria from guinea-pig cerebral cortex and rat heart. Biochem J. 1978 Mar 15;170(3):511–522. doi: 10.1042/bj1700511. [DOI] [PMC free article] [PubMed] [Google Scholar]
- Noth R. H., Mulrow P. J. Serum dopamine beta-hydroxylase as an index of sympathetic nervous system activity in man. Circ Res. 1976 Jan;38(1):1–5. doi: 10.1161/01.res.38.1.1. [DOI] [PubMed] [Google Scholar]
- Patel T. B., Sambasivarao D., Rashed H. M. Role of calcium in synaptosomal substrate oxidation. Arch Biochem Biophys. 1988 Aug 1;264(2):368–375. doi: 10.1016/0003-9861(88)90301-3. [DOI] [PubMed] [Google Scholar]
- Pegg A. E., McCann P. P. Polyamine metabolism and function. Am J Physiol. 1982 Nov;243(5):C212–C221. doi: 10.1152/ajpcell.1982.243.5.C212. [DOI] [PubMed] [Google Scholar]
- Rosenthal M., Jöbsis F. F. Intracellular redox changes in functioning cerebral cortex. II. Effects of direct cortical stimulation. J Neurophysiol. 1971 Sep;34(5):750–762. doi: 10.1152/jn.1971.34.5.750. [DOI] [PubMed] [Google Scholar]
- Rottenberg H., Scarpa A. Calcium uptake and membrane potential in mitochondria. Biochemistry. 1974 Nov 5;13(23):4811–4817. doi: 10.1021/bi00720a020. [DOI] [PubMed] [Google Scholar]
- Rutter G. A., Denton R. M. Regulation of NAD+-linked isocitrate dehydrogenase and 2-oxoglutarate dehydrogenase by Ca2+ ions within toluene-permeabilized rat heart mitochondria. Interactions with regulation by adenine nucleotides and NADH/NAD+ ratios. Biochem J. 1988 May 15;252(1):181–189. doi: 10.1042/bj2520181. [DOI] [PMC free article] [PubMed] [Google Scholar]
- Scott I. D., Nicholls D. G. Energy transduction in intact synaptosomes. Influence of plasma-membrane depolarization on the respiration and membrane potential of internal mitochondria determined in situ. Biochem J. 1980 Jan 15;186(1):21–33. doi: 10.1042/bj1860021. [DOI] [PMC free article] [PubMed] [Google Scholar]
- Segal M., Sagie D. B., Mayevsky A. Metabolic changes induced in rat hippocampal slices by norepinephrine. Brain Res. 1980 Dec 8;202(2):387–399. doi: 10.1016/0006-8993(80)90150-x. [DOI] [PubMed] [Google Scholar]
- Silver R. A., Lamb A. G., Bolsover S. R. Calcium hotspots caused by L-channel clustering promote morphological changes in neuronal growth cones. Nature. 1990 Feb 22;343(6260):751–754. doi: 10.1038/343751a0. [DOI] [PubMed] [Google Scholar]
- Taipale H. T., Kauppinen R. A., Komulainen H. Ruthenium red inhibits the voltage-dependent increase in cytosolic free calcium in cortical synaptosomes from guinea-pig. Biochem Pharmacol. 1989 Apr 1;38(7):1109–1113. doi: 10.1016/0006-2952(89)90256-6. [DOI] [PubMed] [Google Scholar]
- Thayer S. A., Miller R. J. Regulation of the intracellular free calcium concentration in single rat dorsal root ganglion neurones in vitro. J Physiol. 1990 Jun;425:85–115. doi: 10.1113/jphysiol.1990.sp018094. [DOI] [PMC free article] [PubMed] [Google Scholar]
- Vasington F. D., Gazzotti P., Tiozzo R., Carafoli E. The effect of ruthenium red on Ca 2+ transport and respiration in rat liver mitochondria. Biochim Biophys Acta. 1972 Jan 21;256(1):43–54. doi: 10.1016/0005-2728(72)90161-2. [DOI] [PubMed] [Google Scholar]
- Yamada E. W., Huzel N. J. The calcium-binding ATPase inhibitor protein from bovine heart mitochondria. Purification and properties. J Biol Chem. 1988 Aug 15;263(23):11498–11503. [PubMed] [Google Scholar]
- Yamada E. W., Shiffman F. H., Huzel N. J. Ca2+-regulated release of an ATPase inhibitor protein from submitochondrial particles derived from skeletal muscles of the rat. J Biol Chem. 1980 Jan 10;255(1):267–273. [PubMed] [Google Scholar]