Abstract
1. Whole-cell and perforated patch recording techniques were used to examine the activation, deactivation and inactivation of the time-dependent hyperpolarization-activated inward currents (Ih) in isolated superior colliculus-projecting (SCP) neurons from rat primary visual cortex. 2. Examination of inward current waveforms revealed the presence of two kinetically distinct components of Ih: one that activates with a time constant of the order of hundreds of milliseconds, and one that activates with a time constant of the order of seconds. We have termed these Ih,f and Ih,s, to denote the fast and slow components, respectively, of current activation. The time constants of activation of both Ih,f and Ih,s decrease with increasing membrane hyperpolarization. 3. Following the onset of hyperpolarizing voltage steps, a delay is evident prior to time-dependent inward current activation. This delay is voltage dependent and decreases with increasing membrane hyperpolarization. 4. The sigmoidal inward current waveforms are well fitted by the sum of two exponentials in which the faster term, corresponding to the activation of Ih,f, is raised to the power 1.34 +/- 0.26 (mean +/- S.D.). The non-integral exponent suggests that Ih,f activation involves at least two energetically non-equivalent gating transitions prior to channel opening. 5. Over a limited voltage range, tail currents could also be resolved into two distinct components. The faster component, which corresponds to the deactivation of Ih,f, decayed over a single exponential time course with a mean (+/- S.D.) time constant of 355 +/- 161 ms at -70 mV. Ih,s decay also followed a single exponential time course with a mean (+/- S.D.) time constant of 2428 +/- 1285 ms at -70 mV. Both deactivation time constants decreased with increasing depolarization. 6. The separation of inward current activation and deactivation into two distinct components and the lack of correlation between the relative amplitudes of these components suggest that Ih,f and Ih,s reflect the presence of two functionally distinct channel populations. 7. No decrements in time-dependent hyperpolarization-activated inward currents were observed during hyperpolarizations lasting up to 18 s, suggesting that neither Ih,f nor Ih,s inactivates from the open state. In addition, 10 s depolarizations to 0 mV prior to activation did not alter the waveforms of the inward currents activated directly from -40 mV, suggesting that Ih,f and Ih,s also do not inactivate from closed states. 8. The hyperpolarization-activated currents in rat SCP neurons are ideally suited to contribute to the control of the resting membrane potential and input resistance.(ABSTRACT TRUNCATED AT 400 WORDS)
Full text
PDF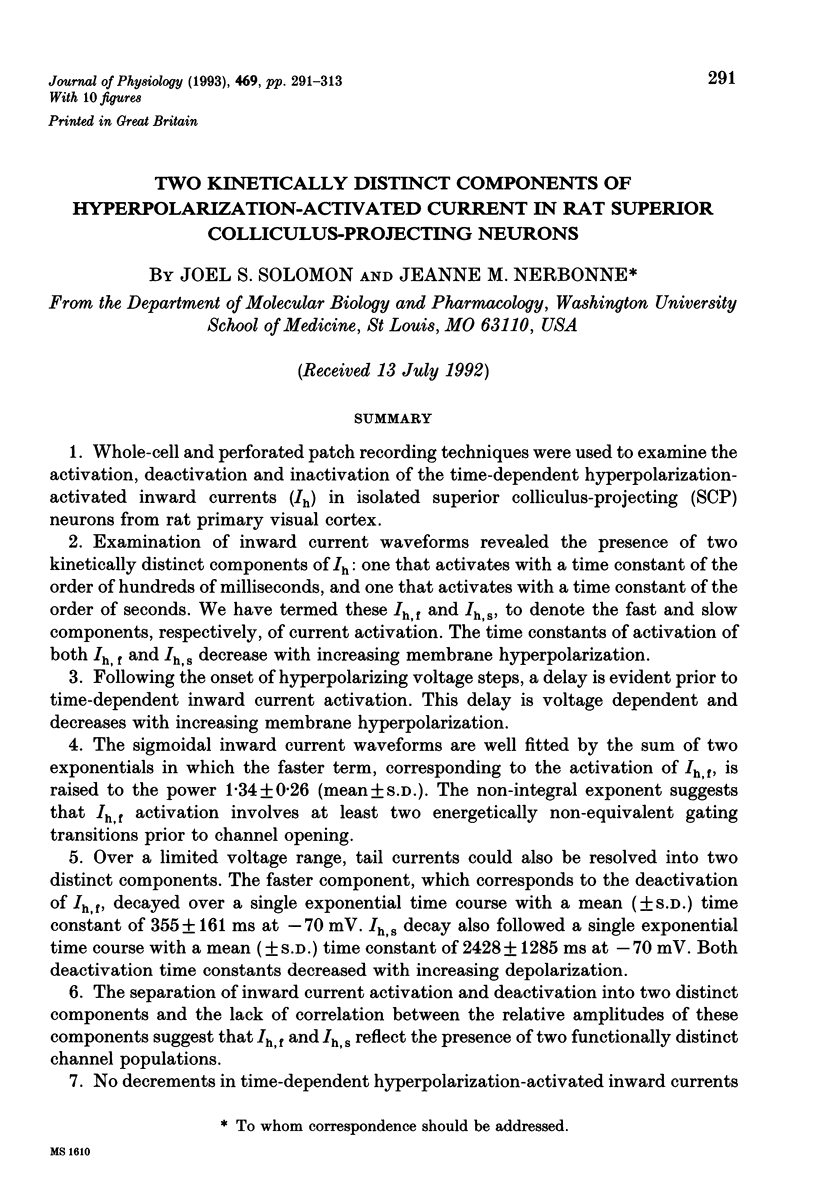
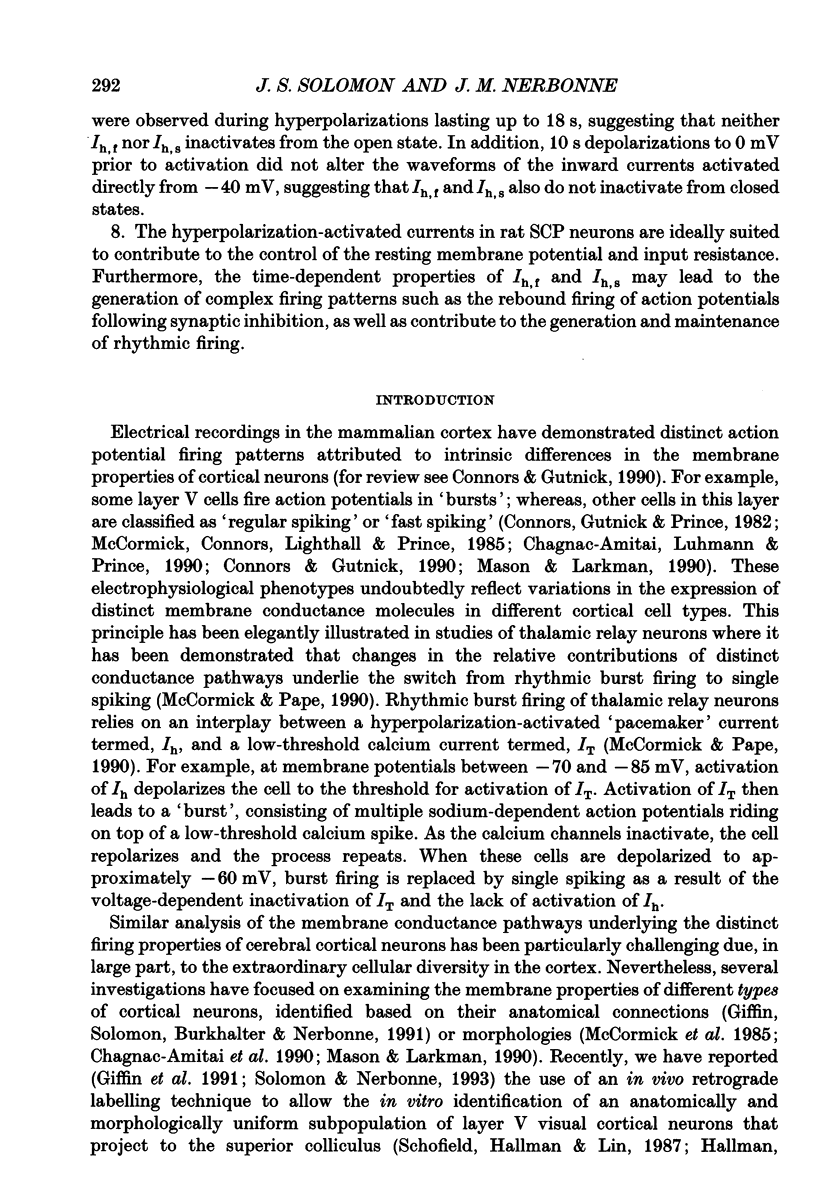
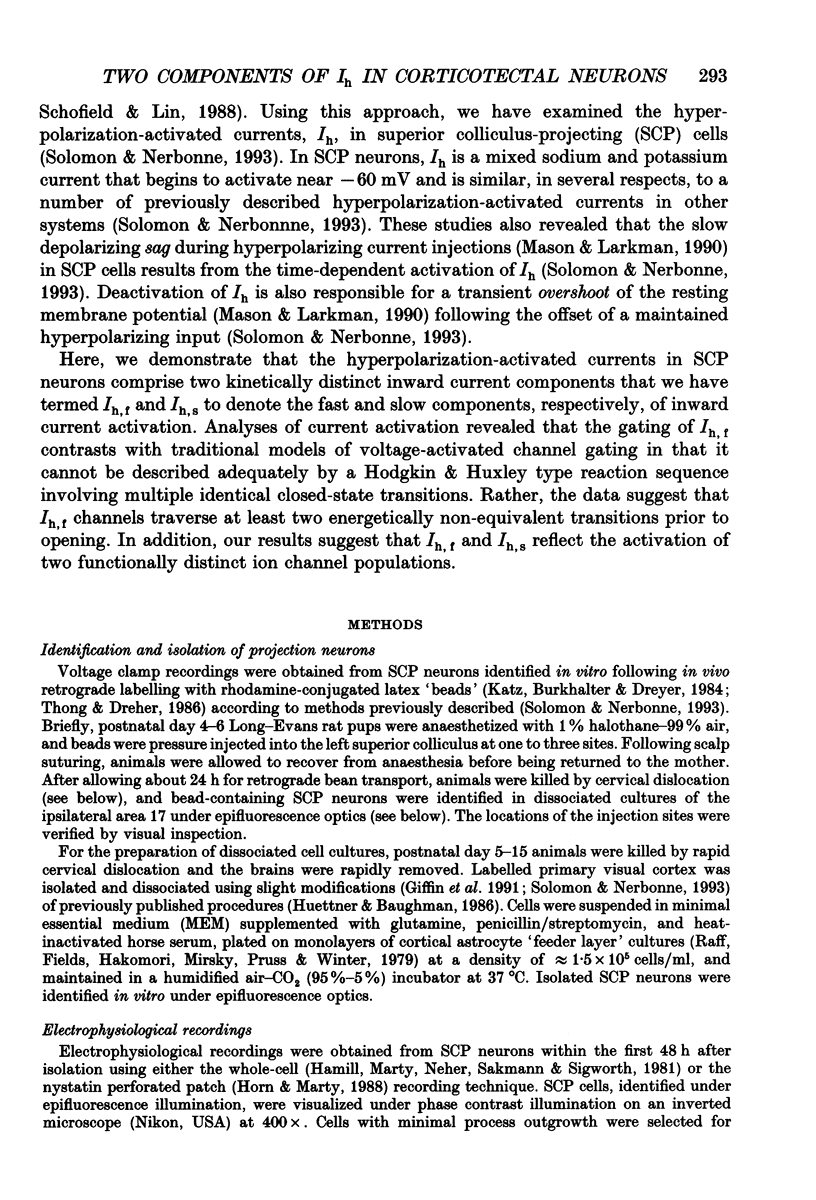
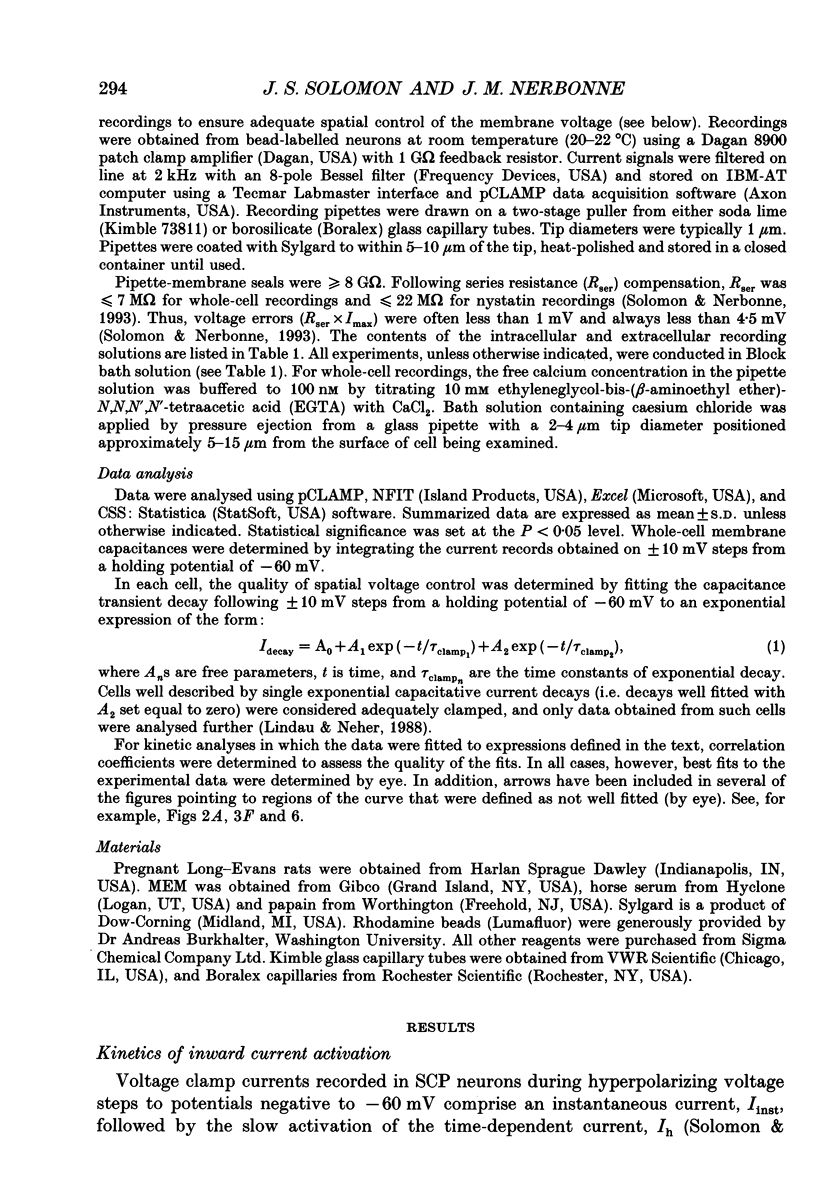
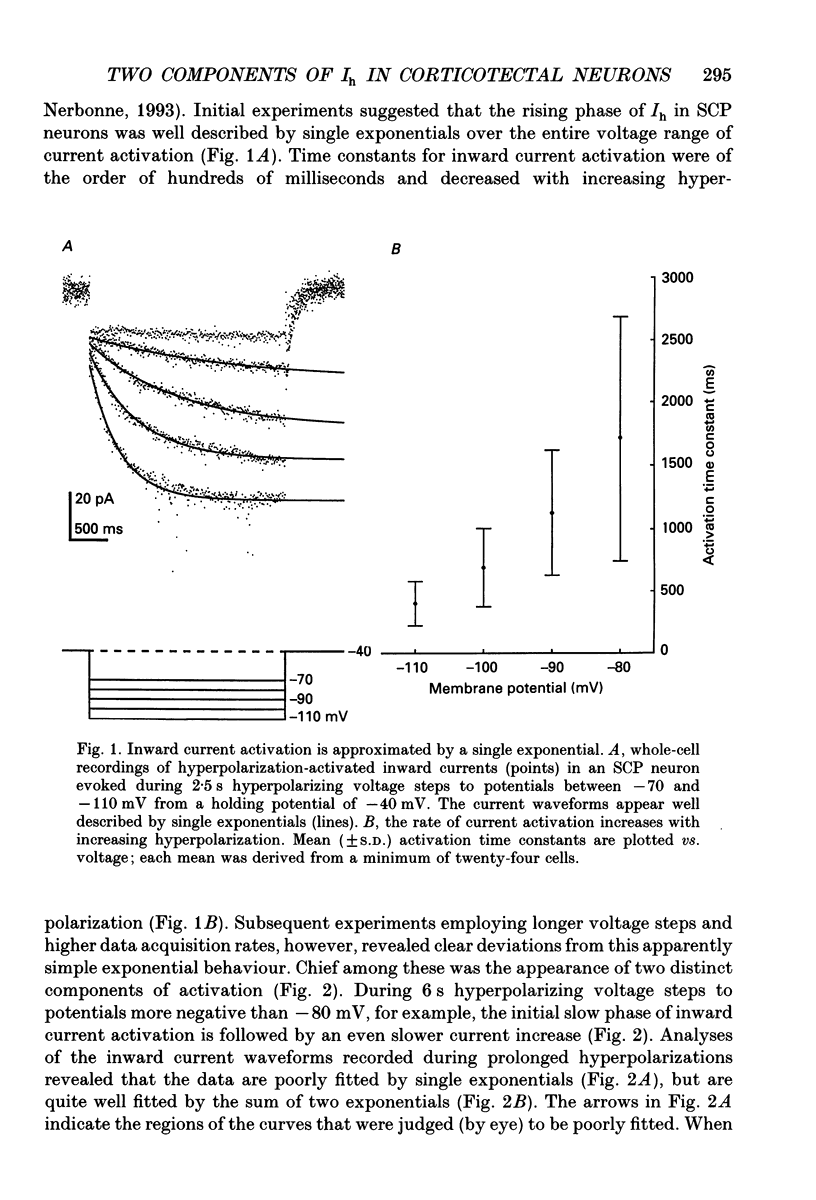
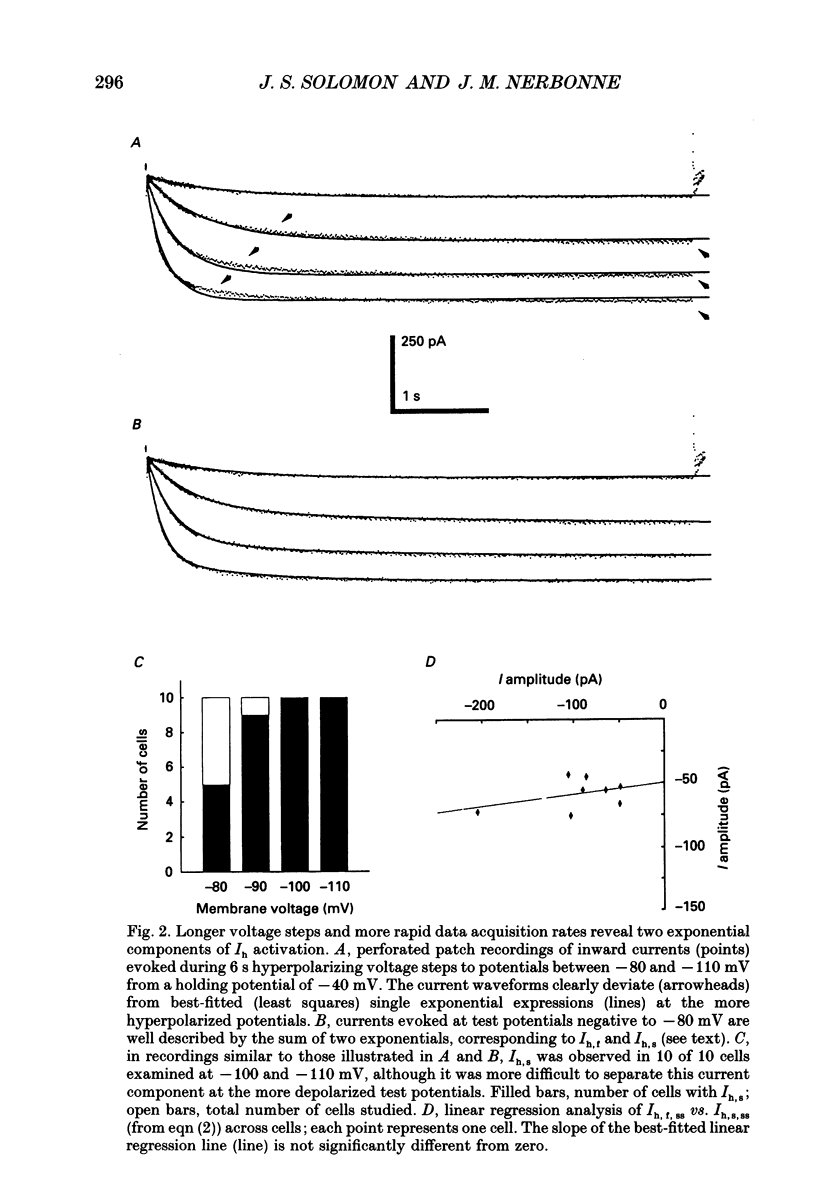
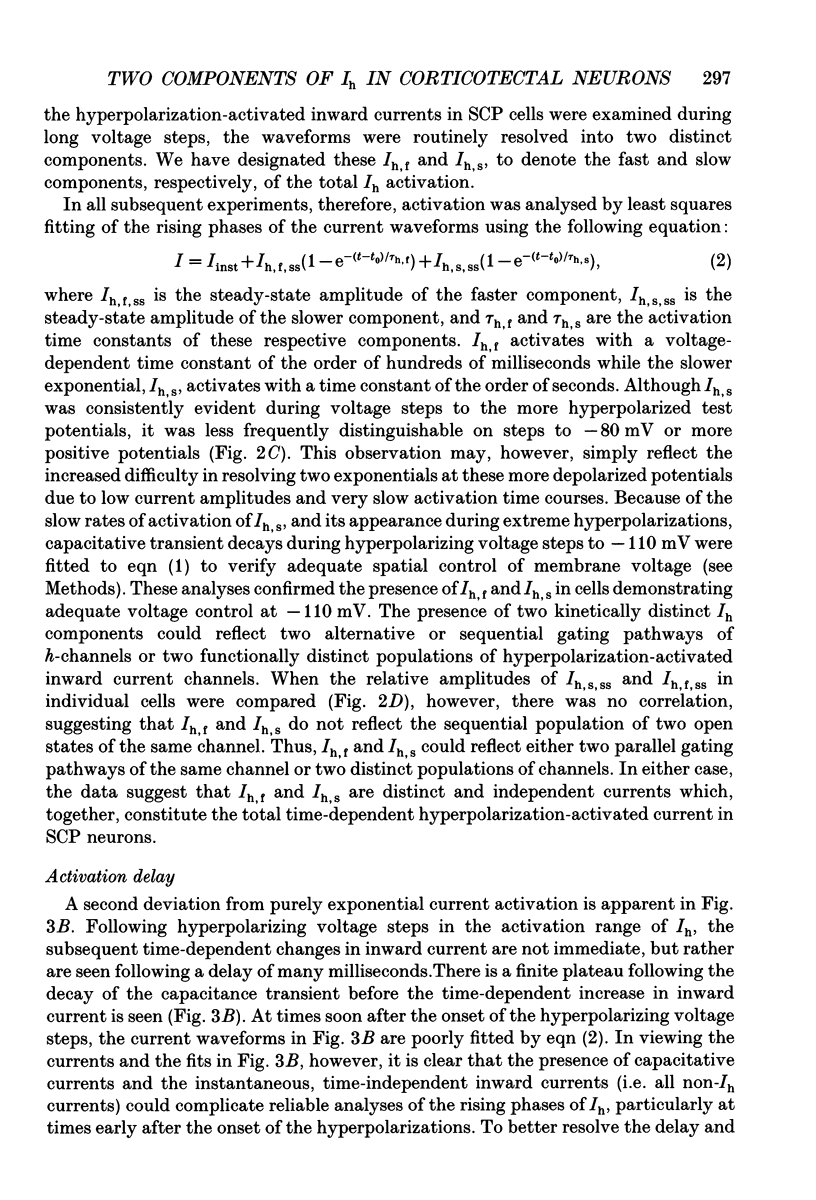
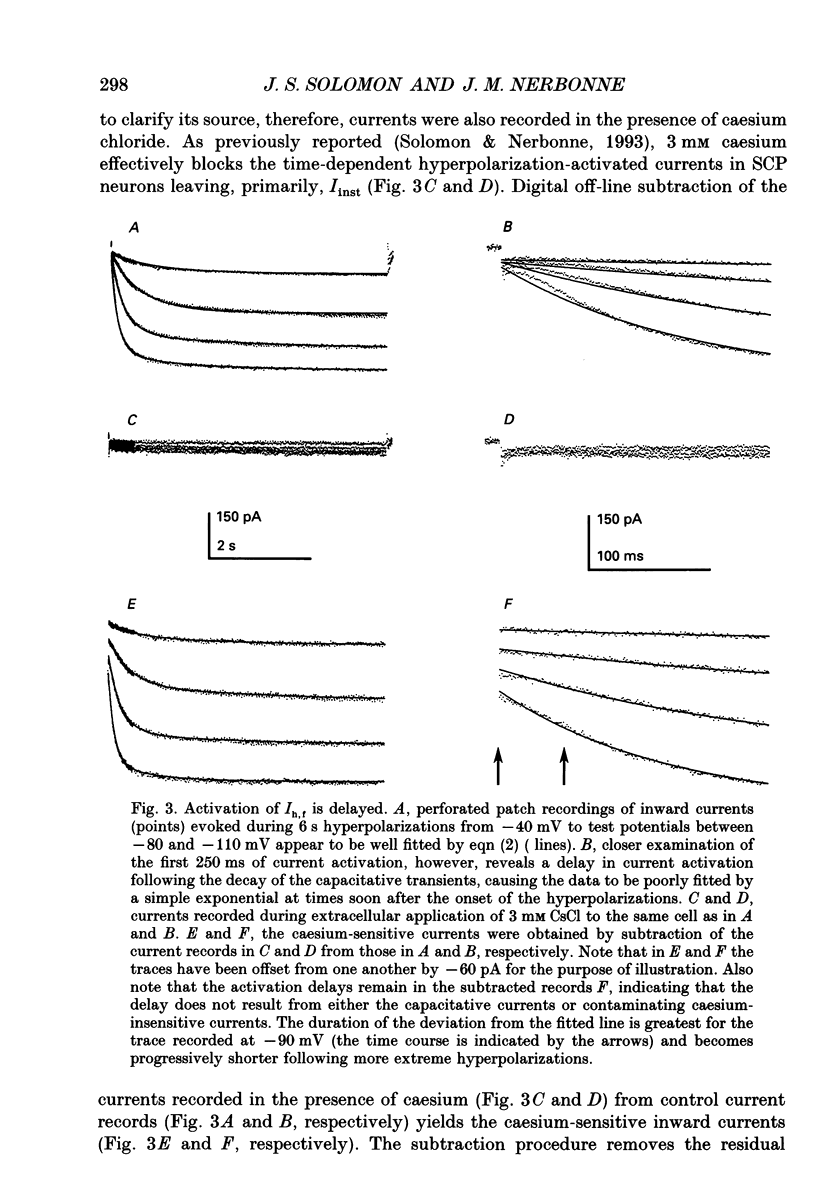
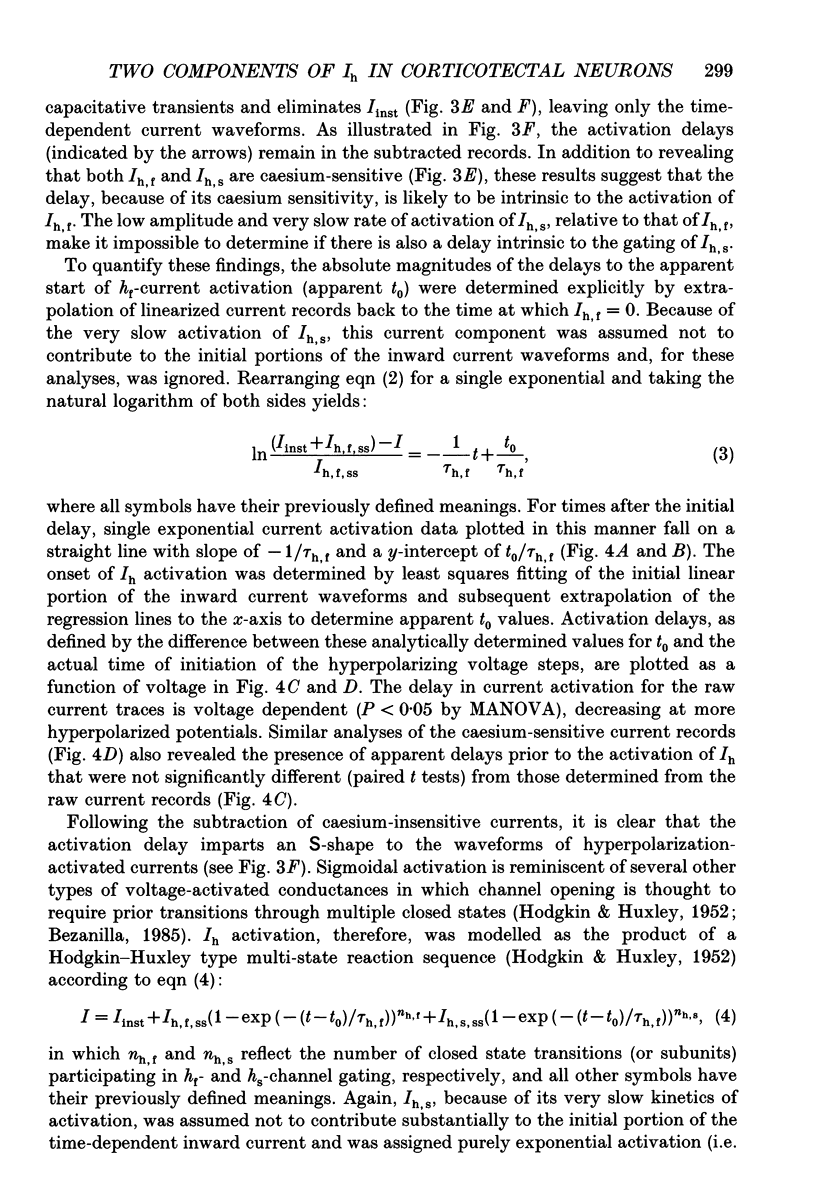
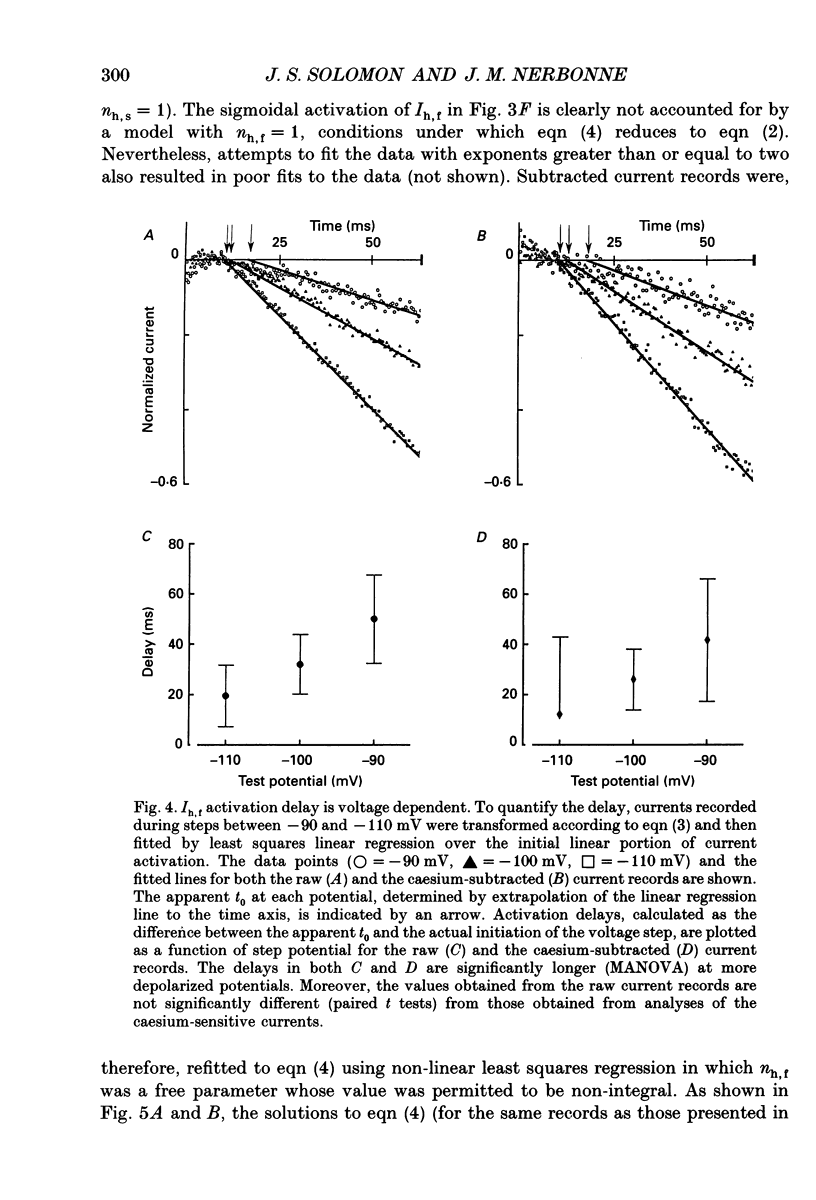
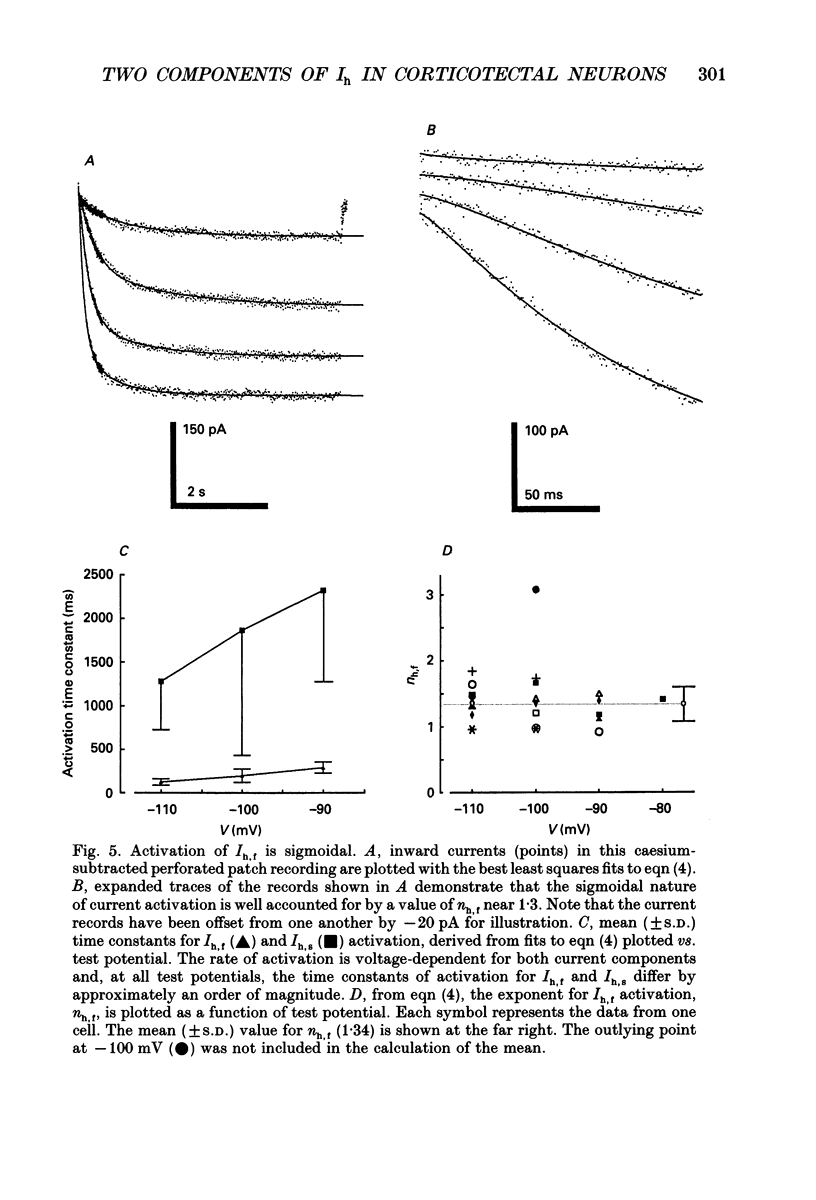
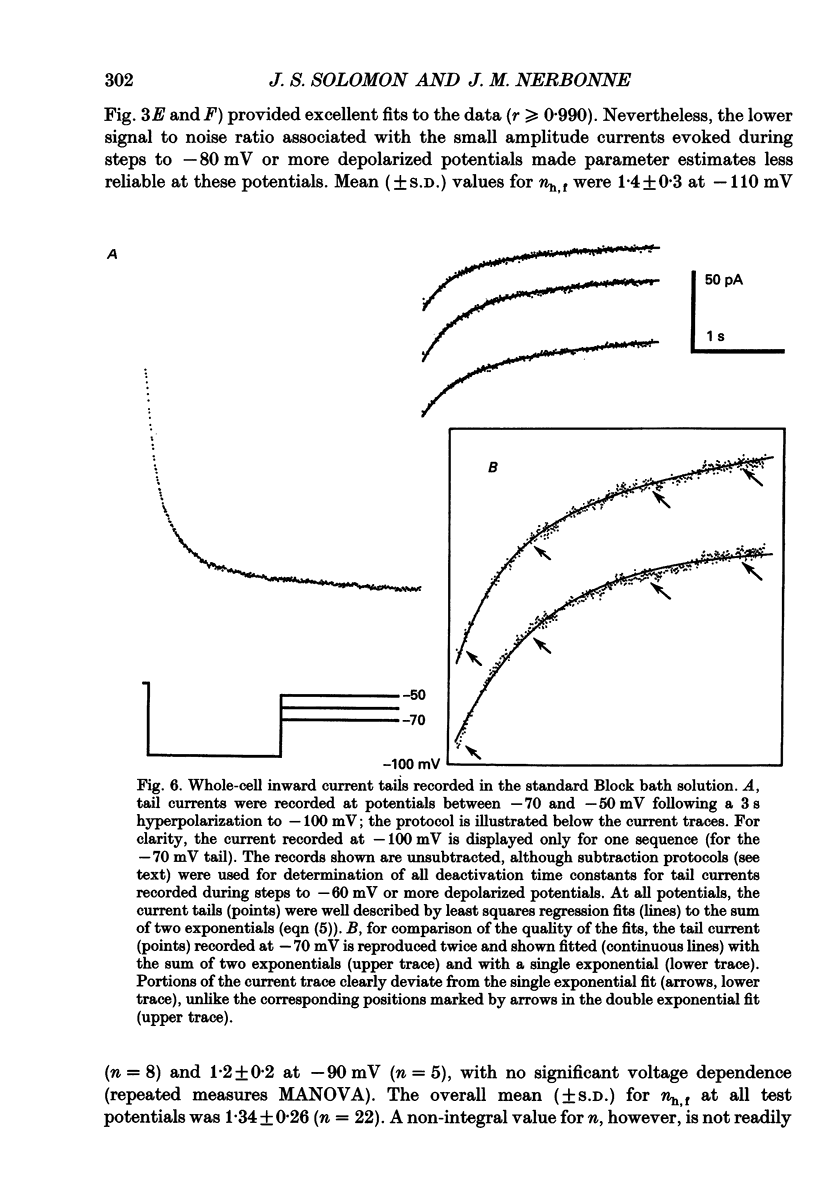
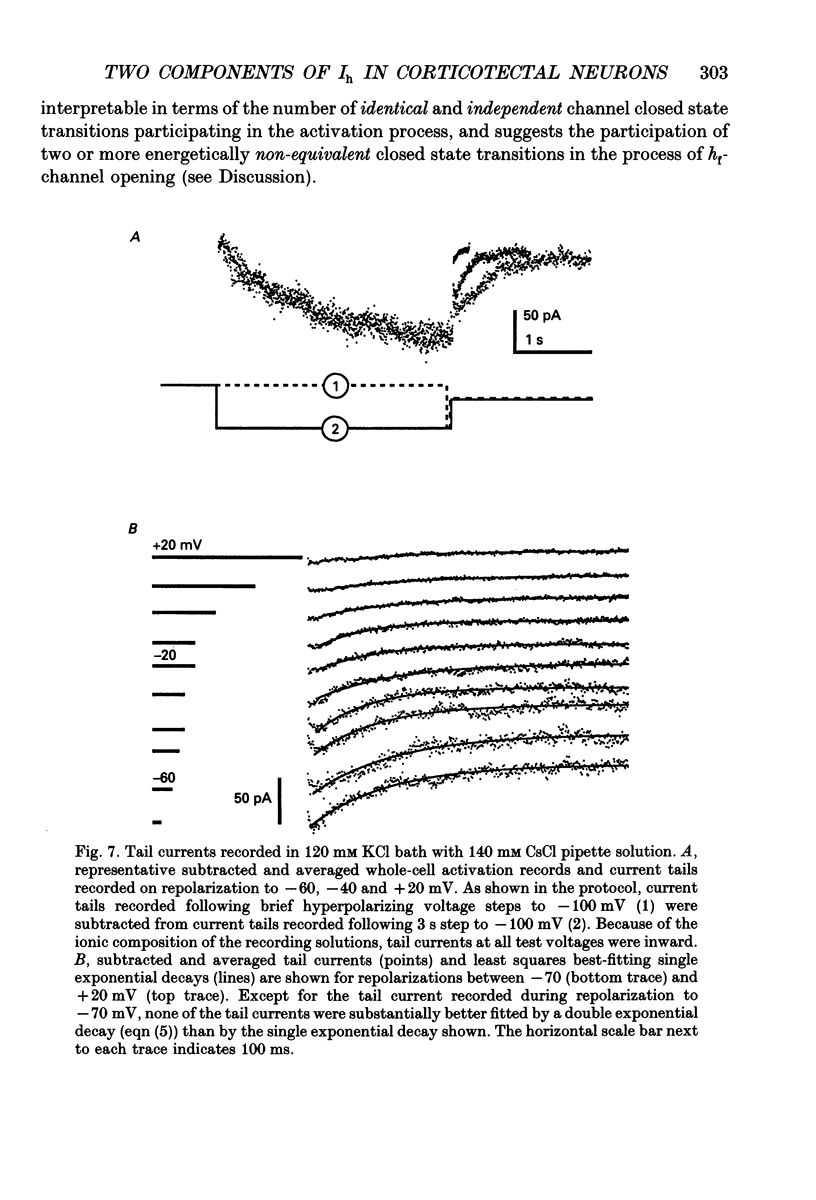
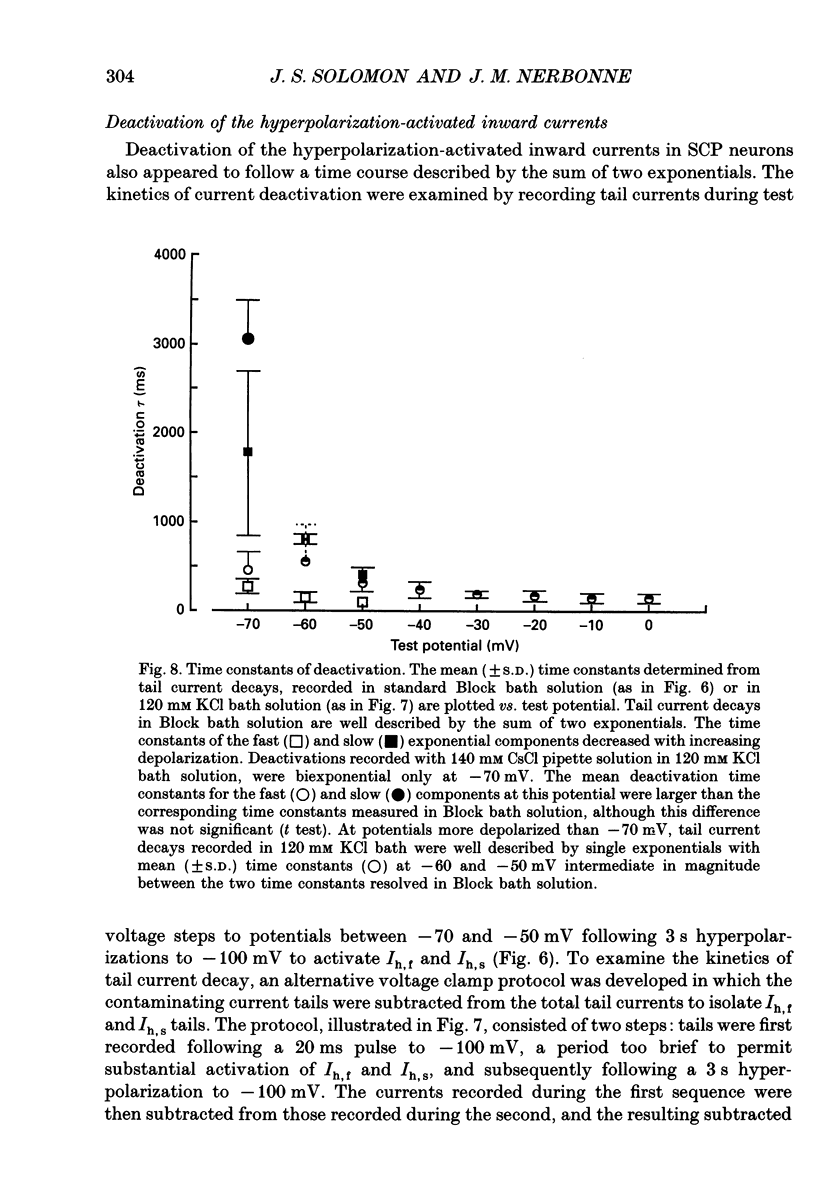
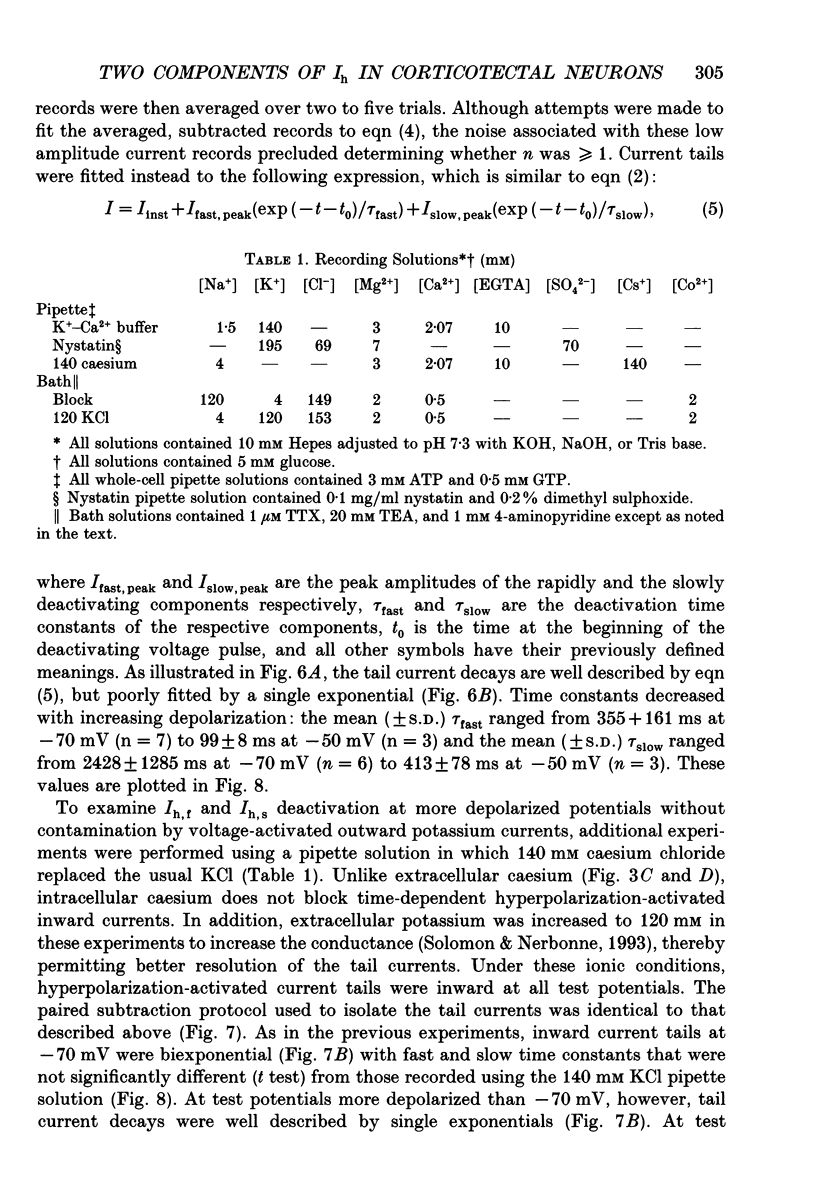
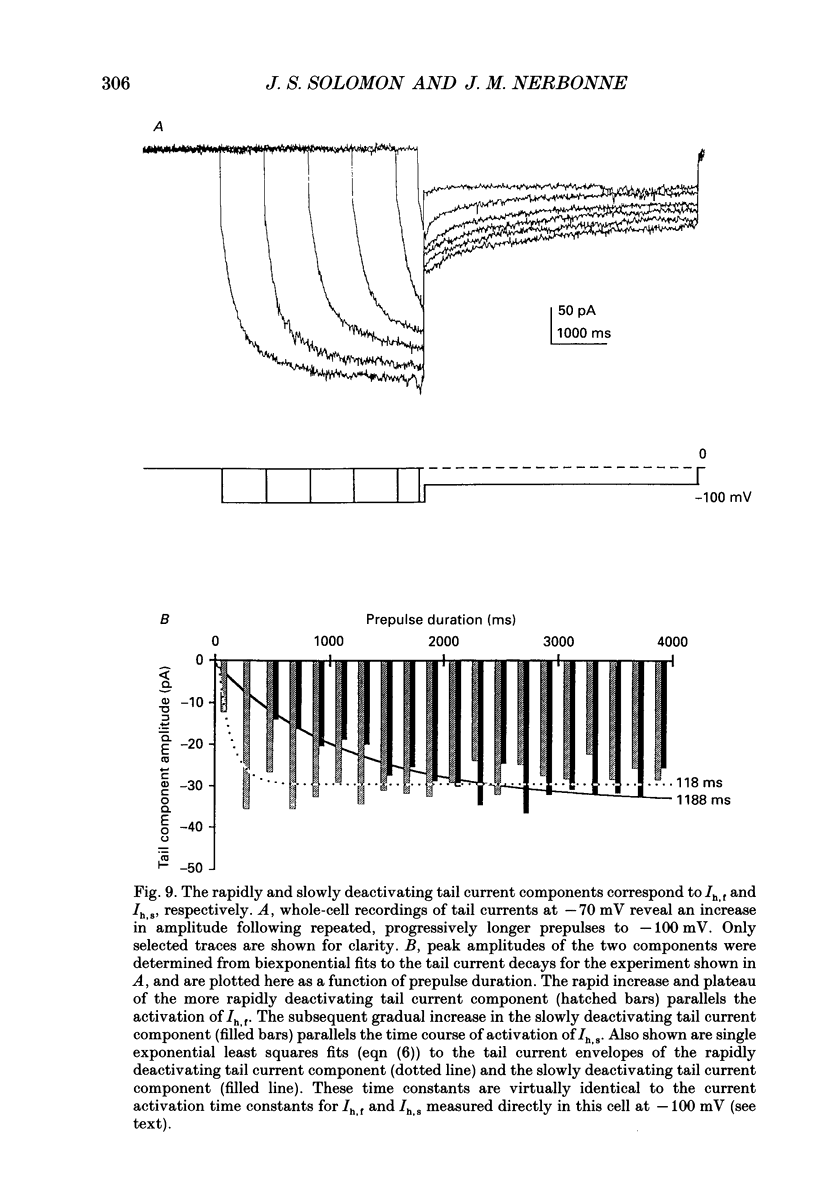
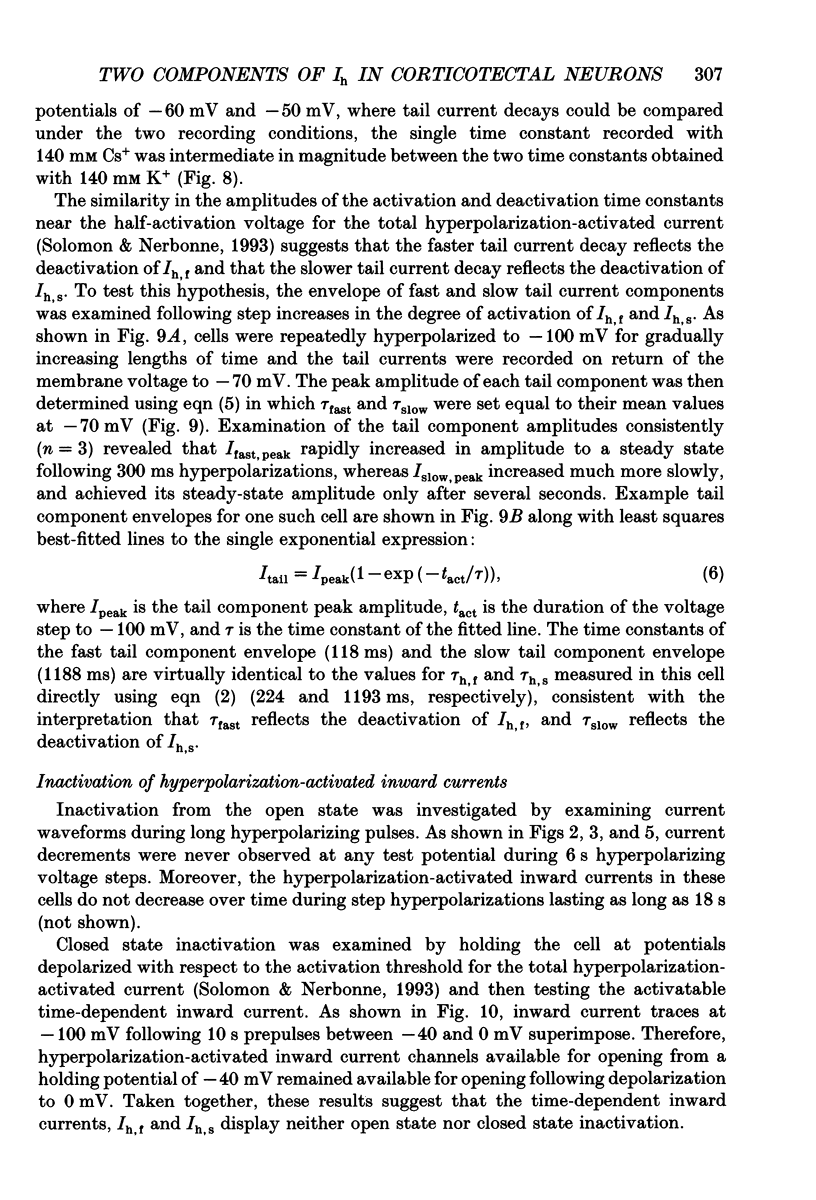
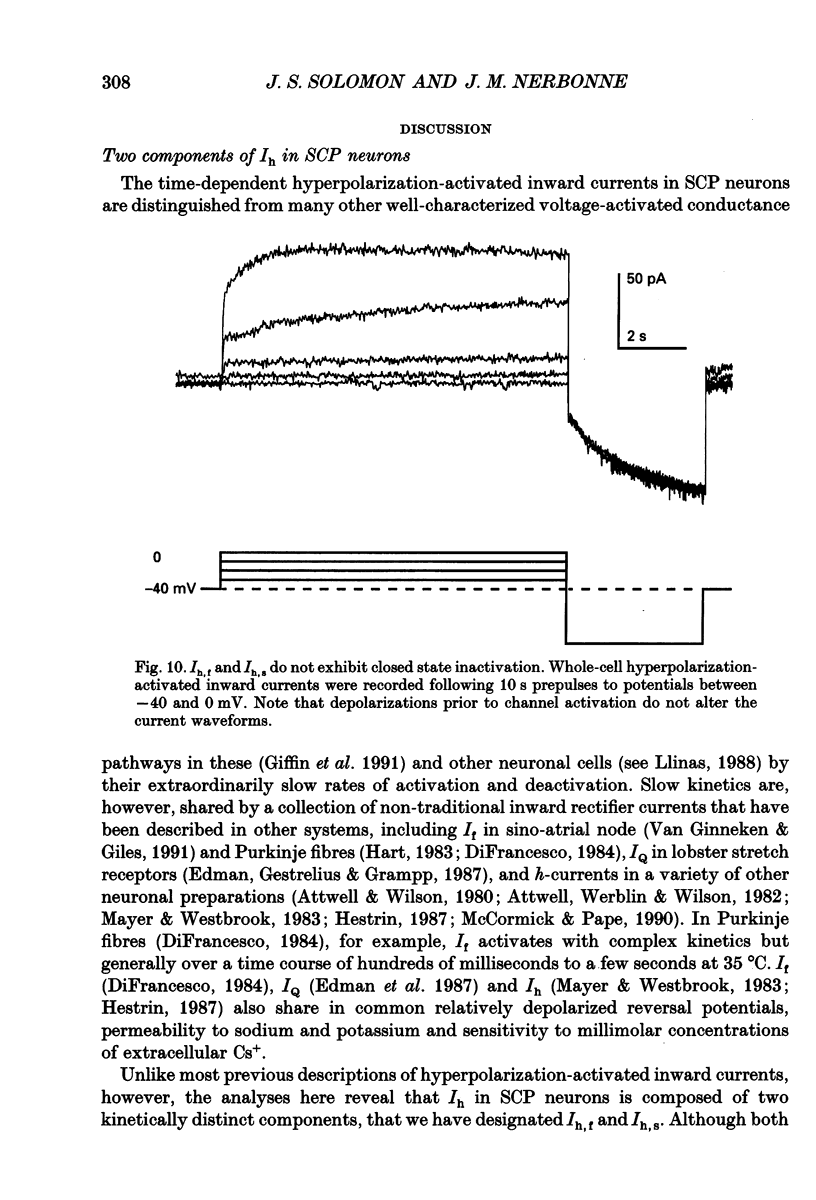
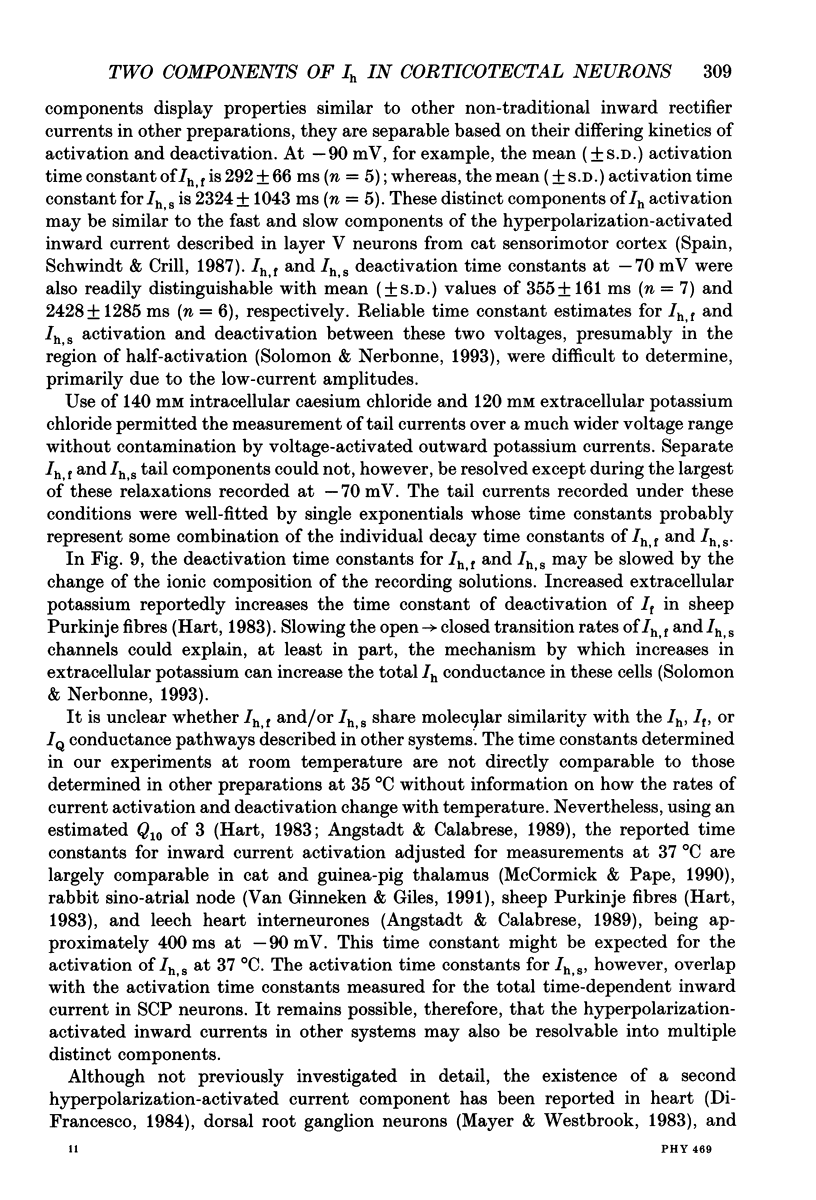
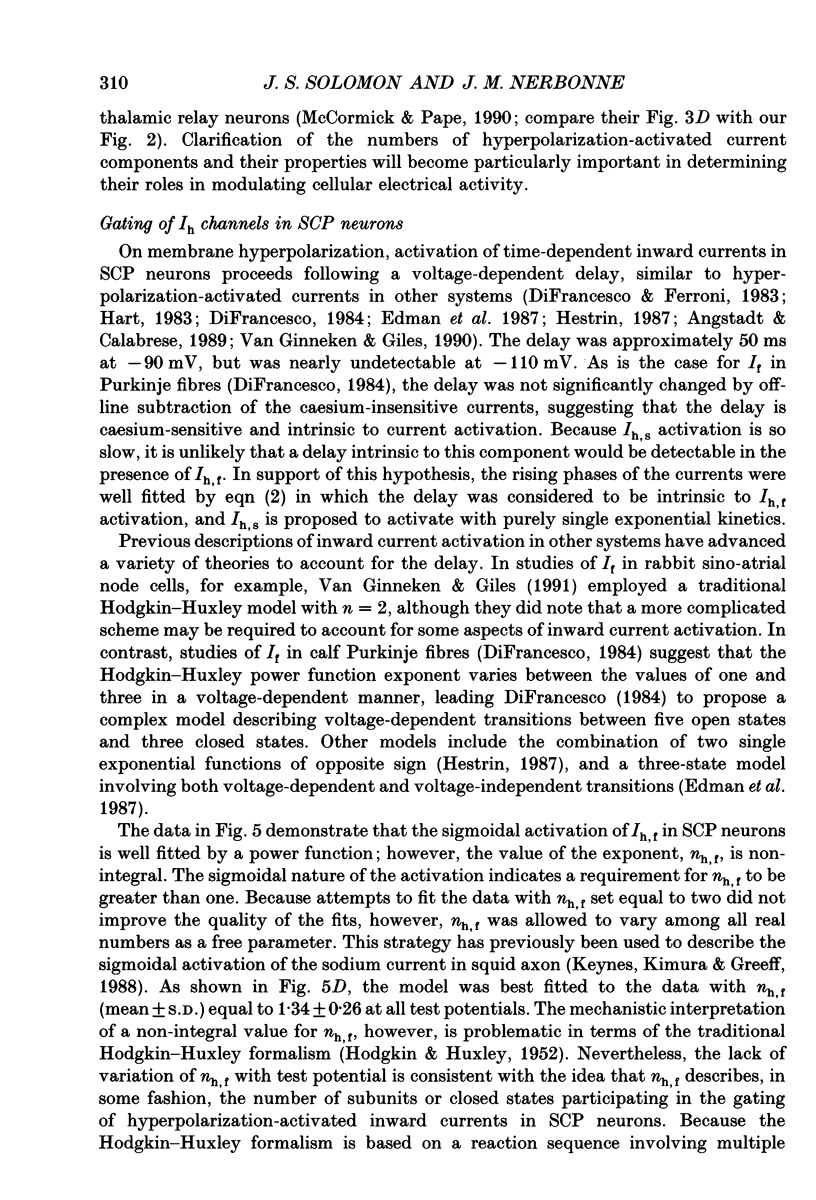
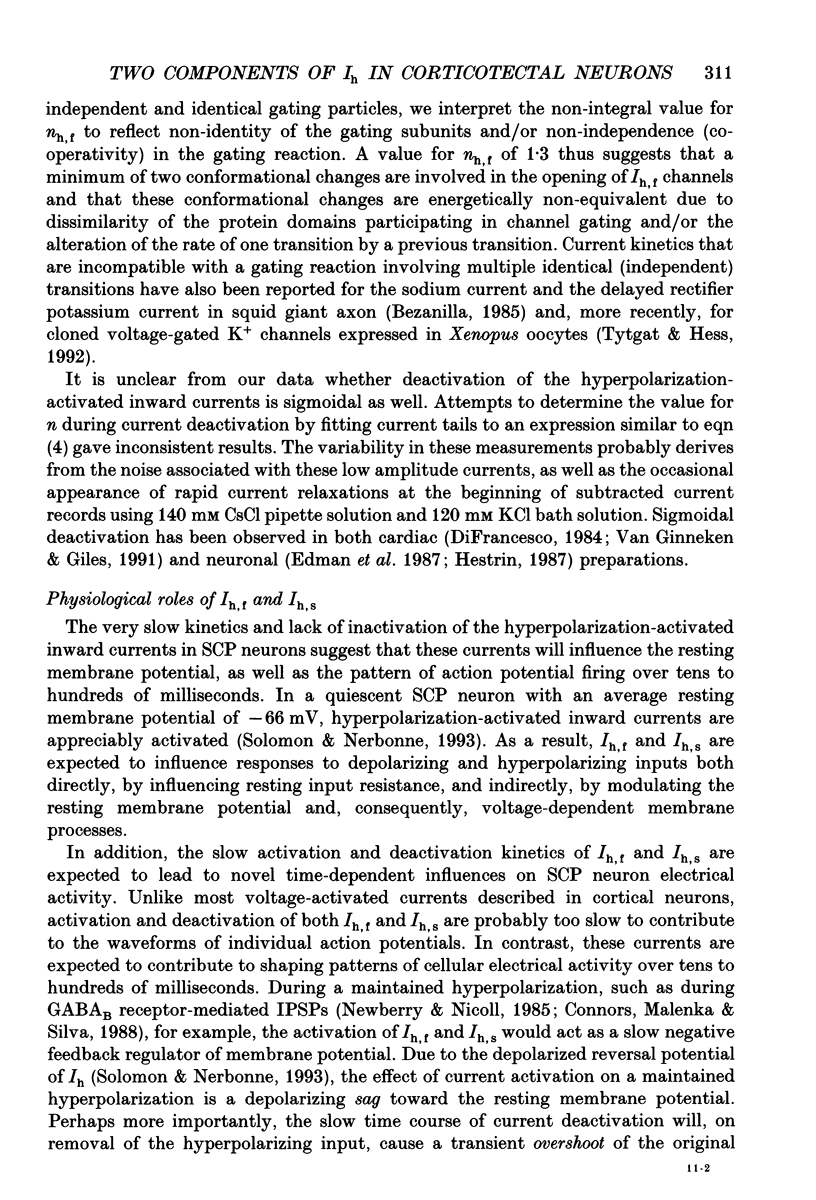
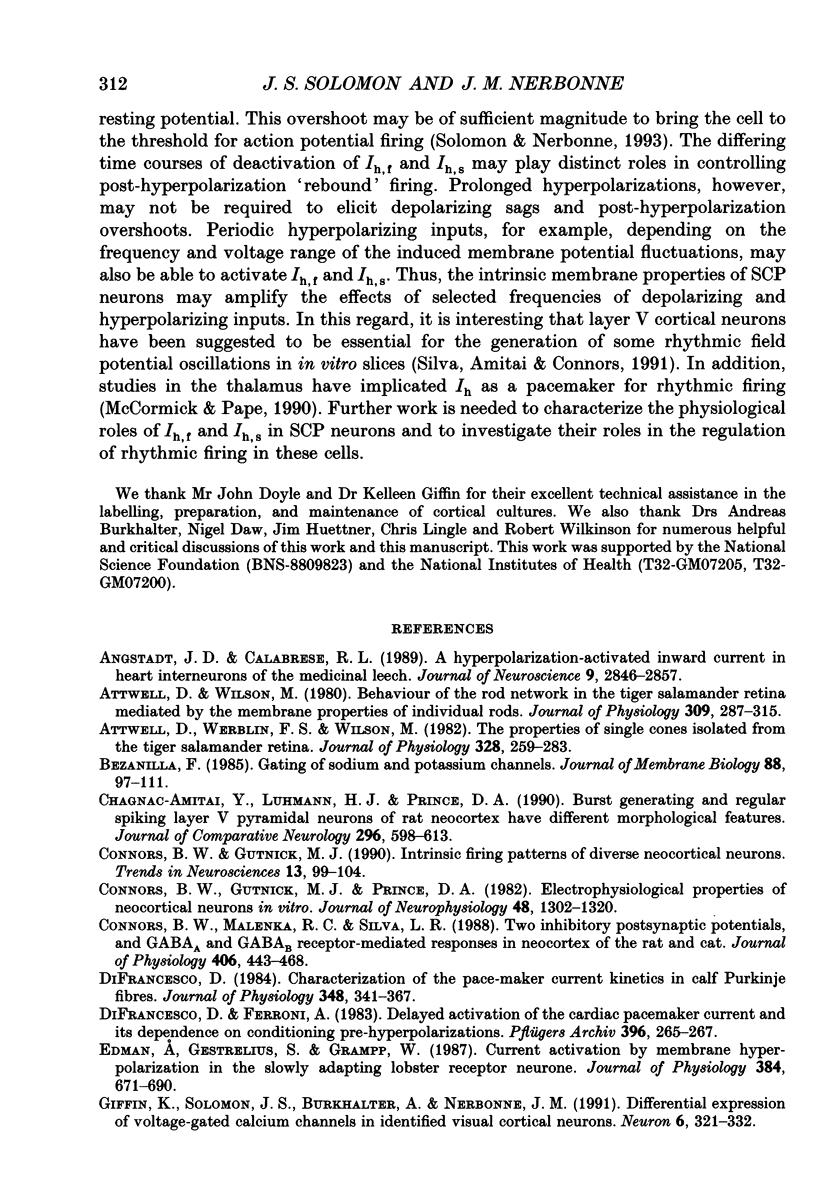
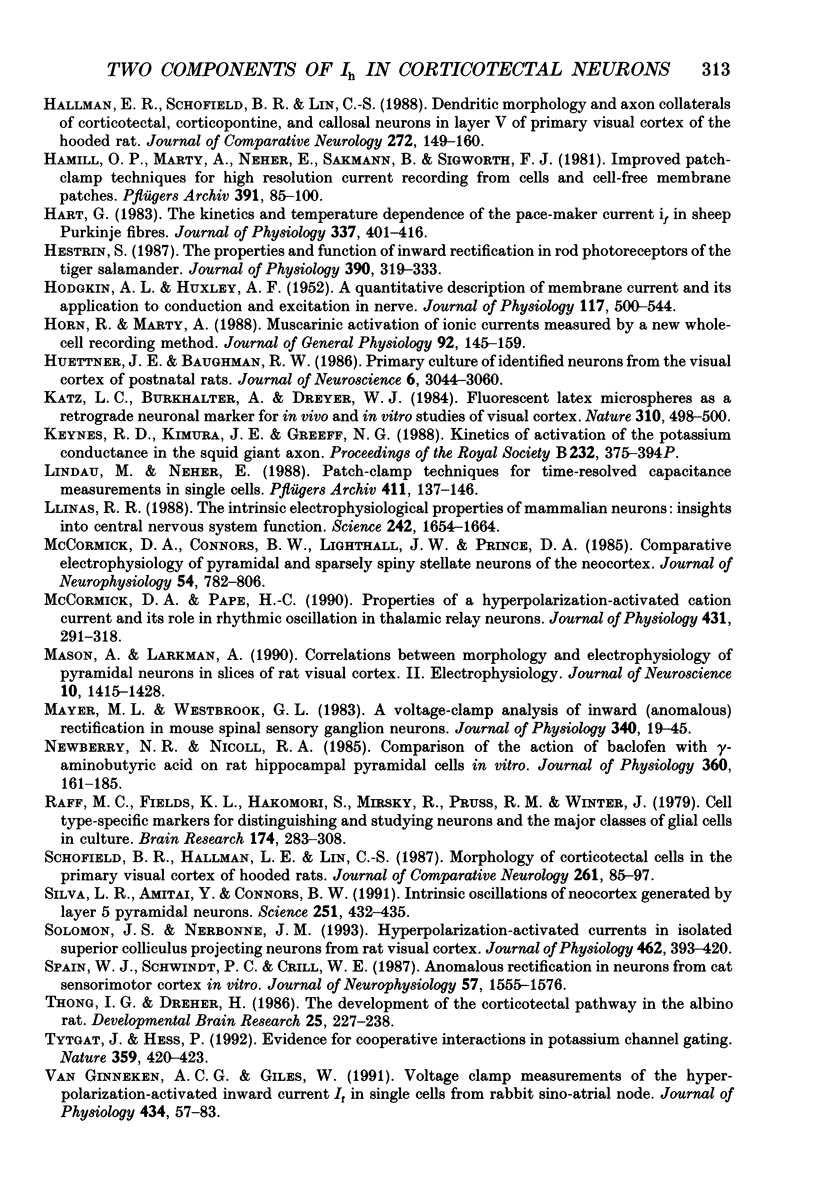
Selected References
These references are in PubMed. This may not be the complete list of references from this article.
- Angstadt J. D., Calabrese R. L. A hyperpolarization-activated inward current in heart interneurons of the medicinal leech. J Neurosci. 1989 Aug;9(8):2846–2857. doi: 10.1523/JNEUROSCI.09-08-02846.1989. [DOI] [PMC free article] [PubMed] [Google Scholar]
- Attwell D., Werblin F. S., Wilson M. The properties of single cones isolated from the tiger salamander retina. J Physiol. 1982 Jul;328:259–283. doi: 10.1113/jphysiol.1982.sp014263. [DOI] [PMC free article] [PubMed] [Google Scholar]
- Attwell D., Wilson M. Behaviour of the rod network in the tiger salamander retina mediated by membrane properties of individual rods. J Physiol. 1980 Dec;309:287–315. doi: 10.1113/jphysiol.1980.sp013509. [DOI] [PMC free article] [PubMed] [Google Scholar]
- Bezanilla F. Gating of sodium and potassium channels. J Membr Biol. 1985;88(2):97–111. doi: 10.1007/BF01868424. [DOI] [PubMed] [Google Scholar]
- Chagnac-Amitai Y., Luhmann H. J., Prince D. A. Burst generating and regular spiking layer 5 pyramidal neurons of rat neocortex have different morphological features. J Comp Neurol. 1990 Jun 22;296(4):598–613. doi: 10.1002/cne.902960407. [DOI] [PubMed] [Google Scholar]
- Connors B. W., Gutnick M. J. Intrinsic firing patterns of diverse neocortical neurons. Trends Neurosci. 1990 Mar;13(3):99–104. doi: 10.1016/0166-2236(90)90185-d. [DOI] [PubMed] [Google Scholar]
- Connors B. W., Gutnick M. J., Prince D. A. Electrophysiological properties of neocortical neurons in vitro. J Neurophysiol. 1982 Dec;48(6):1302–1320. doi: 10.1152/jn.1982.48.6.1302. [DOI] [PubMed] [Google Scholar]
- Connors B. W., Malenka R. C., Silva L. R. Two inhibitory postsynaptic potentials, and GABAA and GABAB receptor-mediated responses in neocortex of rat and cat. J Physiol. 1988 Dec;406:443–468. doi: 10.1113/jphysiol.1988.sp017390. [DOI] [PMC free article] [PubMed] [Google Scholar]
- DiFrancesco D. Characterization of the pace-maker current kinetics in calf Purkinje fibres. J Physiol. 1984 Mar;348:341–367. doi: 10.1113/jphysiol.1984.sp015114. [DOI] [PMC free article] [PubMed] [Google Scholar]
- DiFrancesco D., Ferroni A. Delayed activation of the cardiac pacemaker current and its dependence on conditioning pre-hyperpolarizations. Pflugers Arch. 1983 Mar 1;396(3):265–267. doi: 10.1007/BF00587866. [DOI] [PubMed] [Google Scholar]
- Edman A., Gestrelius S., Grampp W. Current activation by membrane hyperpolarization in the slowly adapting lobster stretch receptor neurone. J Physiol. 1987 Mar;384:671–690. doi: 10.1113/jphysiol.1987.sp016476. [DOI] [PMC free article] [PubMed] [Google Scholar]
- Giffin K., Solomon J. S., Burkhalter A., Nerbonne J. M. Differential expression of voltage-gated calcium channels in identified visual cortical neurons. Neuron. 1991 Mar;6(3):321–332. doi: 10.1016/0896-6273(91)90242-r. [DOI] [PubMed] [Google Scholar]
- HODGKIN A. L., HUXLEY A. F. A quantitative description of membrane current and its application to conduction and excitation in nerve. J Physiol. 1952 Aug;117(4):500–544. doi: 10.1113/jphysiol.1952.sp004764. [DOI] [PMC free article] [PubMed] [Google Scholar]
- Hallman L. E., Schofield B. R., Lin C. S. Dendritic morphology and axon collaterals of corticotectal, corticopontine, and callosal neurons in layer V of primary visual cortex of the hooded rat. J Comp Neurol. 1988 Jun 1;272(1):149–160. doi: 10.1002/cne.902720111. [DOI] [PubMed] [Google Scholar]
- Hamill O. P., Marty A., Neher E., Sakmann B., Sigworth F. J. Improved patch-clamp techniques for high-resolution current recording from cells and cell-free membrane patches. Pflugers Arch. 1981 Aug;391(2):85–100. doi: 10.1007/BF00656997. [DOI] [PubMed] [Google Scholar]
- Hart G. The kinetics and temperature dependence of the pace-maker current if in sheep Purkinje fibres. J Physiol. 1983 Apr;337:401–416. doi: 10.1113/jphysiol.1983.sp014631. [DOI] [PMC free article] [PubMed] [Google Scholar]
- Hestrin S. The properties and function of inward rectification in rod photoreceptors of the tiger salamander. J Physiol. 1987 Sep;390:319–333. doi: 10.1113/jphysiol.1987.sp016703. [DOI] [PMC free article] [PubMed] [Google Scholar]
- Horn R., Marty A. Muscarinic activation of ionic currents measured by a new whole-cell recording method. J Gen Physiol. 1988 Aug;92(2):145–159. doi: 10.1085/jgp.92.2.145. [DOI] [PMC free article] [PubMed] [Google Scholar]
- Huettner J. E., Baughman R. W. Primary culture of identified neurons from the visual cortex of postnatal rats. J Neurosci. 1986 Oct;6(10):3044–3060. doi: 10.1523/JNEUROSCI.06-10-03044.1986. [DOI] [PMC free article] [PubMed] [Google Scholar]
- Katz L. C., Burkhalter A., Dreyer W. J. Fluorescent latex microspheres as a retrograde neuronal marker for in vivo and in vitro studies of visual cortex. Nature. 1984 Aug 9;310(5977):498–500. doi: 10.1038/310498a0. [DOI] [PubMed] [Google Scholar]
- Keynes R. D., Kimura J. E., Greeff N. G. Kinetics of activation of the potassium conductance in the squid giant axon. Proc R Soc Lond B Biol Sci. 1988 Jan 22;232(1269):375–394. doi: 10.1098/rspb.1988.0002. [DOI] [PubMed] [Google Scholar]
- Lindau M., Neher E. Patch-clamp techniques for time-resolved capacitance measurements in single cells. Pflugers Arch. 1988 Feb;411(2):137–146. doi: 10.1007/BF00582306. [DOI] [PubMed] [Google Scholar]
- Llinás R. R. The intrinsic electrophysiological properties of mammalian neurons: insights into central nervous system function. Science. 1988 Dec 23;242(4886):1654–1664. doi: 10.1126/science.3059497. [DOI] [PubMed] [Google Scholar]
- Mason A., Larkman A. Correlations between morphology and electrophysiology of pyramidal neurons in slices of rat visual cortex. II. Electrophysiology. J Neurosci. 1990 May;10(5):1415–1428. doi: 10.1523/JNEUROSCI.10-05-01415.1990. [DOI] [PMC free article] [PubMed] [Google Scholar]
- Mayer M. L., Westbrook G. L. A voltage-clamp analysis of inward (anomalous) rectification in mouse spinal sensory ganglion neurones. J Physiol. 1983 Jul;340:19–45. doi: 10.1113/jphysiol.1983.sp014747. [DOI] [PMC free article] [PubMed] [Google Scholar]
- McCormick D. A., Connors B. W., Lighthall J. W., Prince D. A. Comparative electrophysiology of pyramidal and sparsely spiny stellate neurons of the neocortex. J Neurophysiol. 1985 Oct;54(4):782–806. doi: 10.1152/jn.1985.54.4.782. [DOI] [PubMed] [Google Scholar]
- McCormick D. A., Pape H. C. Properties of a hyperpolarization-activated cation current and its role in rhythmic oscillation in thalamic relay neurones. J Physiol. 1990 Dec;431:291–318. doi: 10.1113/jphysiol.1990.sp018331. [DOI] [PMC free article] [PubMed] [Google Scholar]
- Newberry N. R., Nicoll R. A. Comparison of the action of baclofen with gamma-aminobutyric acid on rat hippocampal pyramidal cells in vitro. J Physiol. 1985 Mar;360:161–185. doi: 10.1113/jphysiol.1985.sp015610. [DOI] [PMC free article] [PubMed] [Google Scholar]
- Raff M. C., Fields K. L., Hakomori S. I., Mirsky R., Pruss R. M., Winter J. Cell-type-specific markers for distinguishing and studying neurons and the major classes of glial cells in culture. Brain Res. 1979 Oct 5;174(2):283–308. doi: 10.1016/0006-8993(79)90851-5. [DOI] [PubMed] [Google Scholar]
- Schofield B. R., Hallman L. E., Lin C. S. Morphology of corticotectal cells in the primary visual cortex of hooded rats. J Comp Neurol. 1987 Jul 1;261(1):85–97. doi: 10.1002/cne.902610107. [DOI] [PubMed] [Google Scholar]
- Silva L. R., Amitai Y., Connors B. W. Intrinsic oscillations of neocortex generated by layer 5 pyramidal neurons. Science. 1991 Jan 25;251(4992):432–435. doi: 10.1126/science.1824881. [DOI] [PubMed] [Google Scholar]
- Solomon J. S., Nerbonne J. M. Hyperpolarization-activated currents in isolated superior colliculus-projecting neurons from rat visual cortex. J Physiol. 1993 Mar;462:393–420. doi: 10.1113/jphysiol.1993.sp019561. [DOI] [PMC free article] [PubMed] [Google Scholar]
- Spain W. J., Schwindt P. C., Crill W. E. Anomalous rectification in neurons from cat sensorimotor cortex in vitro. J Neurophysiol. 1987 May;57(5):1555–1576. doi: 10.1152/jn.1987.57.5.1555. [DOI] [PubMed] [Google Scholar]
- Thong I. G., Dreher B. The development of the corticotectal pathway in the albino rat. Brain Res. 1986 Mar;390(2):227–238. doi: 10.1016/s0006-8993(86)80231-1. [DOI] [PubMed] [Google Scholar]
- Tytgat J., Hess P. Evidence for cooperative interactions in potassium channel gating. Nature. 1992 Oct 1;359(6394):420–423. doi: 10.1038/359420a0. [DOI] [PubMed] [Google Scholar]
- van Ginneken A. C., Giles W. Voltage clamp measurements of the hyperpolarization-activated inward current I(f) in single cells from rabbit sino-atrial node. J Physiol. 1991 Mar;434:57–83. doi: 10.1113/jphysiol.1991.sp018459. [DOI] [PMC free article] [PubMed] [Google Scholar]