Abstract
Sensitivity of enzymes to inhibition by thiol-reactive reagents is often presented as evidence for the possible involvement of cysteine residues in substrate binding and catalysis or to highlight possible important differences in structure and mechanism between closely related enzymes. The primary phenotypic distinction between the enterobacterial type II chloramphenicol acetyltransferase (CATII; typified by the enzyme encoded by the incW transmissible plasmid pSa) and the CATI and CATIII variants is the greatly enhanced susceptibility of CATII to inactivation by thiol-specific modifying reagents. Determination of the nucleotide sequence of the gene, catII, present on pSa and that of a related determinant, catIIH, isolated from Haemophilus influenzae indicates that sensitivity to such reagents cannot be due to the presence of additional reactive cysteine residues in CATII. Comparative analysis of the inactivation of CATII and CATIII by 5,5'-dithiobis-(2-nitrobenzoic acid) (DTNB), 4,4'-dithiodipyridine (DTDP) and methyl methanethiosulphonate (MMTS) suggests that (i) inactivation occurs as a result of chemical modification of the same residue (Cys-31) in each enzyme, (ii) reagents that inactivate via a pseudo-first-order process (DTNB and DTDP) appear to bind with a greater affinity to CATII, and (iii) the intrinsic reactivity of Cys-31 in CATII greatly exceeds that of the corresponding residue in CATIII. The results lead to the conclusion that a striking difference in chemical reactivity of a unique and conserved thiol group between closely related enzyme variants may not be easily explained even when a high-resolution tertiary structure is available for one of them. Plausible explanations include more favourable access of reagents to Cys-31 in CATII or an enhanced reactivity of its thiol group imposed by the side chains of residues that are not in immediate contact with it.
Full text
PDF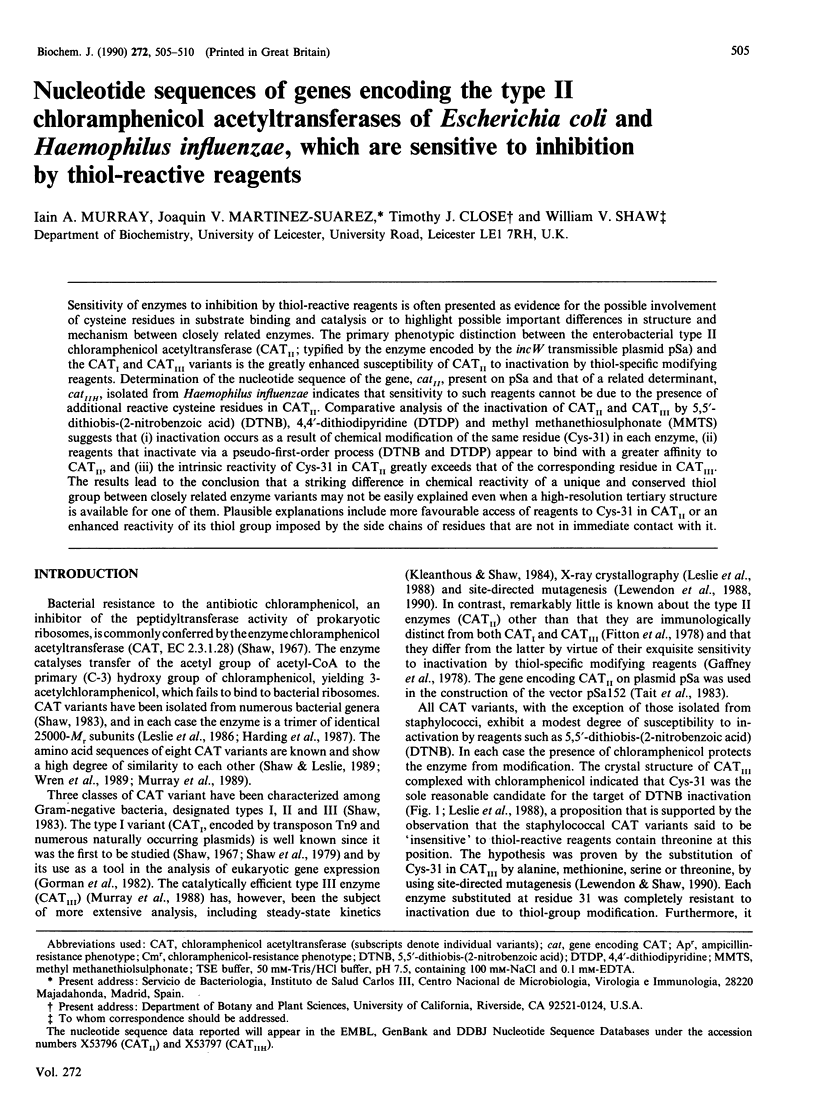
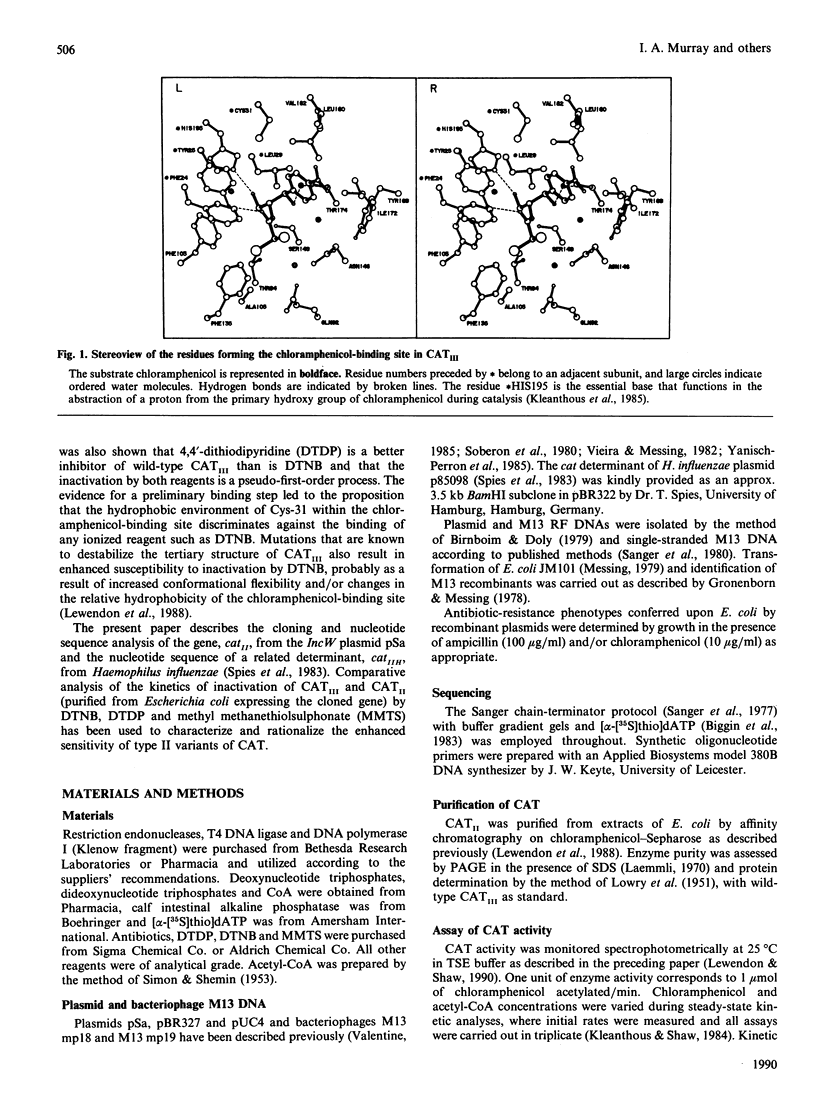
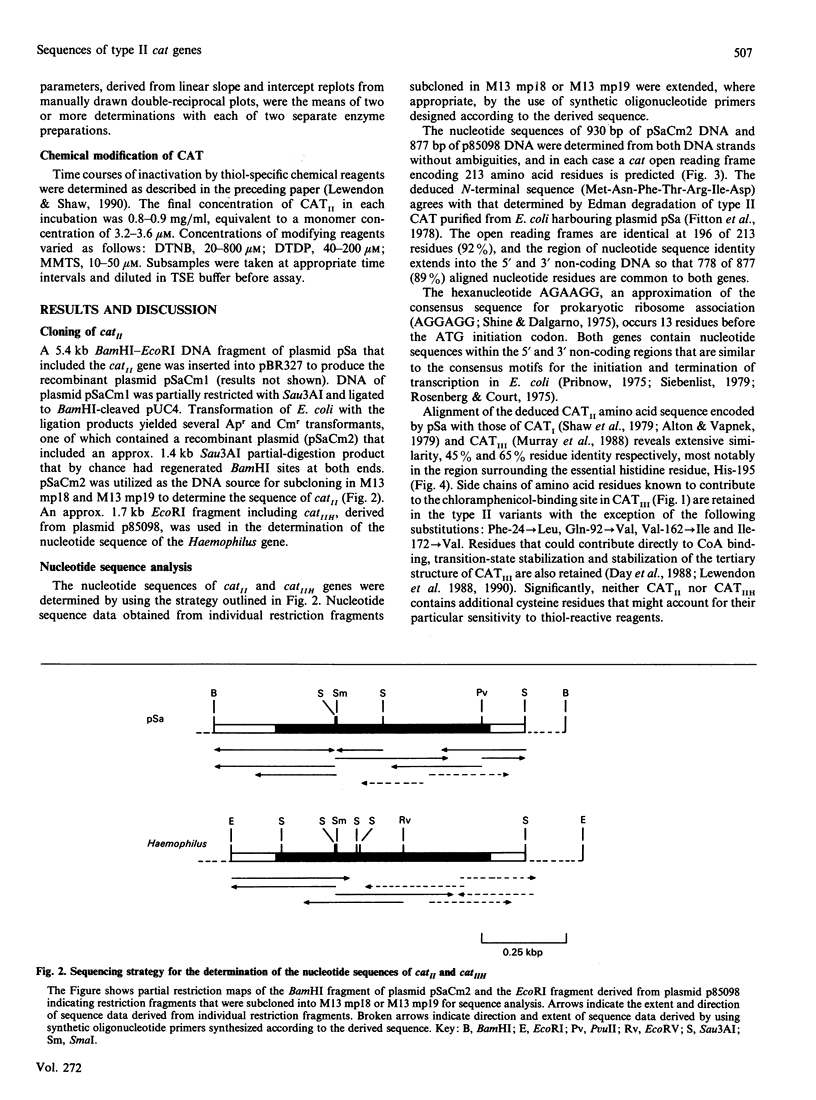

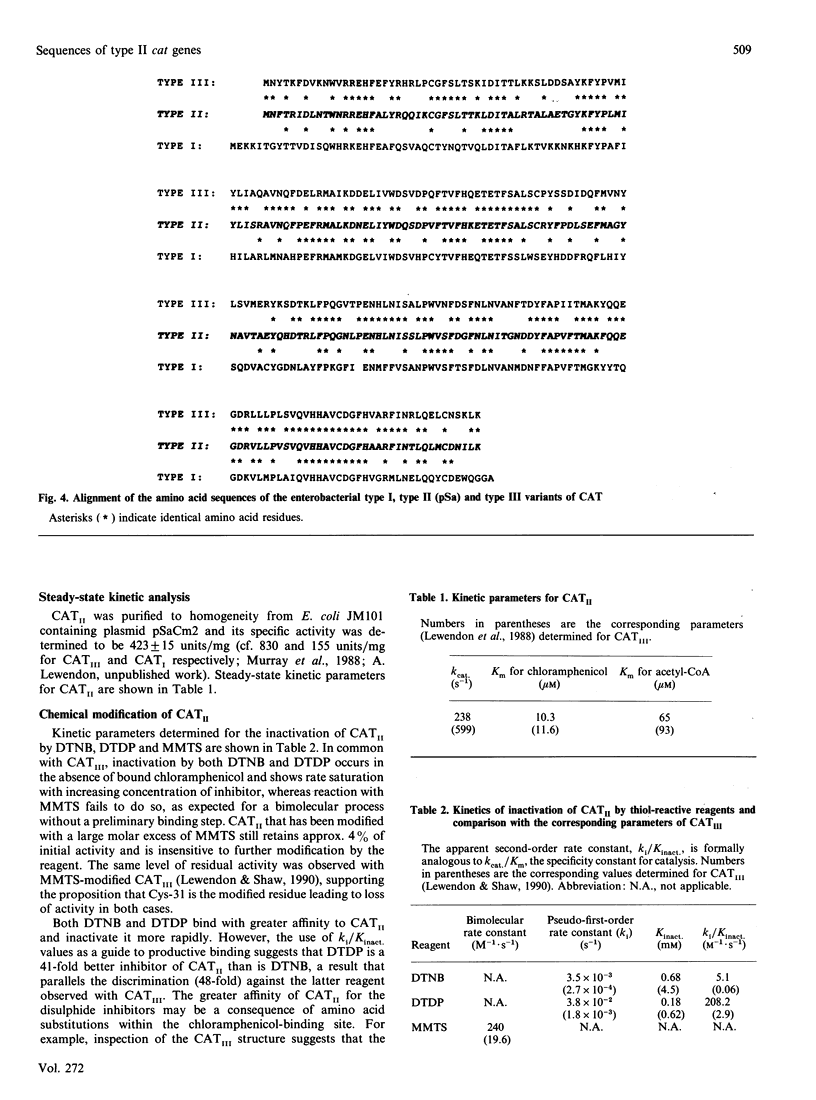
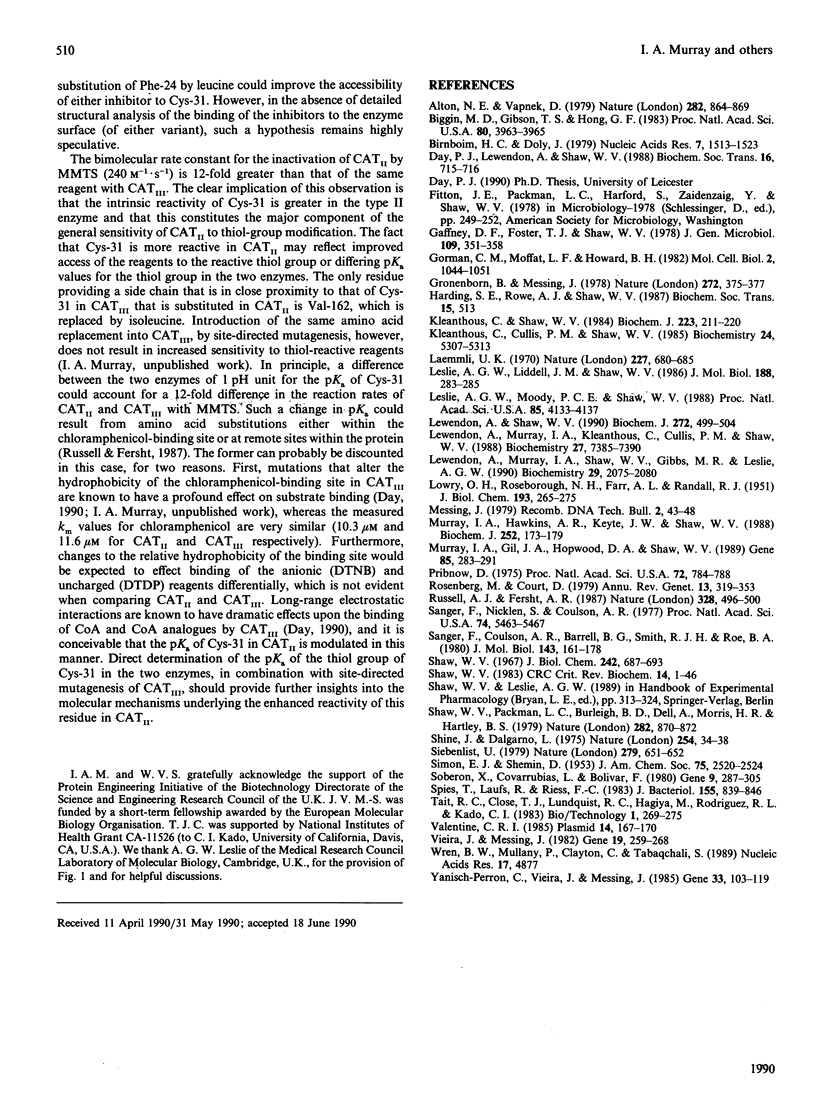
Selected References
These references are in PubMed. This may not be the complete list of references from this article.
- Alton N. K., Vapnek D. Nucleotide sequence analysis of the chloramphenicol resistance transposon Tn9. Nature. 1979 Dec 20;282(5741):864–869. doi: 10.1038/282864a0. [DOI] [PubMed] [Google Scholar]
- Biggin M. D., Gibson T. J., Hong G. F. Buffer gradient gels and 35S label as an aid to rapid DNA sequence determination. Proc Natl Acad Sci U S A. 1983 Jul;80(13):3963–3965. doi: 10.1073/pnas.80.13.3963. [DOI] [PMC free article] [PubMed] [Google Scholar]
- Birnboim H. C., Doly J. A rapid alkaline extraction procedure for screening recombinant plasmid DNA. Nucleic Acids Res. 1979 Nov 24;7(6):1513–1523. doi: 10.1093/nar/7.6.1513. [DOI] [PMC free article] [PubMed] [Google Scholar]
- Gorman C. M., Moffat L. F., Howard B. H. Recombinant genomes which express chloramphenicol acetyltransferase in mammalian cells. Mol Cell Biol. 1982 Sep;2(9):1044–1051. doi: 10.1128/mcb.2.9.1044. [DOI] [PMC free article] [PubMed] [Google Scholar]
- Gronenborn B., Messing J. Methylation of single-stranded DNA in vitro introduces new restriction endonuclease cleavage sites. Nature. 1978 Mar 23;272(5651):375–377. doi: 10.1038/272375a0. [DOI] [PubMed] [Google Scholar]
- Kleanthous C., Cullis P. M., Shaw W. V. 3-(Bromoacetyl)chloramphenicol, an active site directed inhibitor for chloramphenicol acetyltransferase. Biochemistry. 1985 Sep 24;24(20):5307–5313. doi: 10.1021/bi00341a006. [DOI] [PubMed] [Google Scholar]
- Kleanthous C., Shaw W. V. Analysis of the mechanism of chloramphenicol acetyltransferase by steady-state kinetics. Evidence for a ternary-complex mechanism. Biochem J. 1984 Oct 1;223(1):211–220. doi: 10.1042/bj2230211. [DOI] [PMC free article] [PubMed] [Google Scholar]
- LOWRY O. H., ROSEBROUGH N. J., FARR A. L., RANDALL R. J. Protein measurement with the Folin phenol reagent. J Biol Chem. 1951 Nov;193(1):265–275. [PubMed] [Google Scholar]
- Laemmli U. K. Cleavage of structural proteins during the assembly of the head of bacteriophage T4. Nature. 1970 Aug 15;227(5259):680–685. doi: 10.1038/227680a0. [DOI] [PubMed] [Google Scholar]
- Leslie A. G., Liddell J. M., Shaw W. V. Crystallization of a type III chloramphenicol acetyl transferase. J Mol Biol. 1986 Mar 20;188(2):283–285. doi: 10.1016/0022-2836(86)90310-4. [DOI] [PubMed] [Google Scholar]
- Leslie A. G., Moody P. C., Shaw W. V. Structure of chloramphenicol acetyltransferase at 1.75-A resolution. Proc Natl Acad Sci U S A. 1988 Jun;85(12):4133–4137. doi: 10.1073/pnas.85.12.4133. [DOI] [PMC free article] [PubMed] [Google Scholar]
- Lewendon A., Murray I. A., Kleanthous C., Cullis P. M., Shaw W. V. Substitutions in the active site of chloramphenicol acetyltransferase: role of a conserved aspartate. Biochemistry. 1988 Sep 20;27(19):7385–7390. doi: 10.1021/bi00419a032. [DOI] [PubMed] [Google Scholar]
- Lewendon A., Murray I. A., Shaw W. V., Gibbs M. R., Leslie A. G. Evidence for transition-state stabilization by serine-148 in the catalytic mechanism of chloramphenicol acetyltransferase. Biochemistry. 1990 Feb 27;29(8):2075–2080. doi: 10.1021/bi00460a016. [DOI] [PubMed] [Google Scholar]
- Lewendon A., Shaw W. V. Elimination of a reactive thiol group from the active site of chloramphenicol acetyltransferase. Biochem J. 1990 Dec 1;272(2):499–504. doi: 10.1042/bj2720499. [DOI] [PMC free article] [PubMed] [Google Scholar]
- Murray I. A., Gil J. A., Hopwood D. A., Shaw W. V. Nucleotide sequence of the chloramphenicol acetyltransferase gene of Streptomyces acrimycini. Gene. 1989 Dec 28;85(2):283–291. doi: 10.1016/0378-1119(89)90420-4. [DOI] [PubMed] [Google Scholar]
- Murray I. A., Hawkins A. R., Keyte J. W., Shaw W. V. Nucleotide sequence analysis and overexpression of the gene encoding a type III chloramphenicol acetyltransferase. Biochem J. 1988 May 15;252(1):173–179. doi: 10.1042/bj2520173. [DOI] [PMC free article] [PubMed] [Google Scholar]
- Pribnow D. Nucleotide sequence of an RNA polymerase binding site at an early T7 promoter. Proc Natl Acad Sci U S A. 1975 Mar;72(3):784–788. doi: 10.1073/pnas.72.3.784. [DOI] [PMC free article] [PubMed] [Google Scholar]
- Rosenberg M., Court D. Regulatory sequences involved in the promotion and termination of RNA transcription. Annu Rev Genet. 1979;13:319–353. doi: 10.1146/annurev.ge.13.120179.001535. [DOI] [PubMed] [Google Scholar]
- Russell A. J., Fersht A. R. Rational modification of enzyme catalysis by engineering surface charge. Nature. 1987 Aug 6;328(6130):496–500. doi: 10.1038/328496a0. [DOI] [PubMed] [Google Scholar]
- Sanger F., Coulson A. R., Barrell B. G., Smith A. J., Roe B. A. Cloning in single-stranded bacteriophage as an aid to rapid DNA sequencing. J Mol Biol. 1980 Oct 25;143(2):161–178. doi: 10.1016/0022-2836(80)90196-5. [DOI] [PubMed] [Google Scholar]
- Sanger F., Nicklen S., Coulson A. R. DNA sequencing with chain-terminating inhibitors. Proc Natl Acad Sci U S A. 1977 Dec;74(12):5463–5467. doi: 10.1073/pnas.74.12.5463. [DOI] [PMC free article] [PubMed] [Google Scholar]
- Shaw W. V. Chloramphenicol acetyltransferase: enzymology and molecular biology. CRC Crit Rev Biochem. 1983;14(1):1–46. doi: 10.3109/10409238309102789. [DOI] [PubMed] [Google Scholar]
- Shaw W. V., Packman L. C., Burleigh B. D., Dell A., Morris H. R., Hartley B. S. Primary structure of a chloramphenicol acetyltransferase specified by R plasmids. Nature. 1979 Dec 20;282(5741):870–872. doi: 10.1038/282870a0. [DOI] [PubMed] [Google Scholar]
- Shaw W. V. The enzymatic acetylation of chloramphenicol by extracts of R factor-resistant Escherichia coli. J Biol Chem. 1967 Feb 25;242(4):687–693. [PubMed] [Google Scholar]
- Shine J., Dalgarno L. Determinant of cistron specificity in bacterial ribosomes. Nature. 1975 Mar 6;254(5495):34–38. doi: 10.1038/254034a0. [DOI] [PubMed] [Google Scholar]
- Siebenlist U. RNA polymerase unwinds an 11-base pair segment of a phage T7 promoter. Nature. 1979 Jun 14;279(5714):651–652. doi: 10.1038/279651a0. [DOI] [PubMed] [Google Scholar]
- Soberon X., Covarrubias L., Bolivar F. Construction and characterization of new cloning vehicles. IV. Deletion derivatives of pBR322 and pBR325. Gene. 1980 May;9(3-4):287–305. doi: 10.1016/0378-1119(90)90328-o. [DOI] [PubMed] [Google Scholar]
- Spies T., Laufs R., Riess F. C. Amplification of resistance genes in Haemophilus influenzae plasmids. J Bacteriol. 1983 Aug;155(2):839–846. doi: 10.1128/jb.155.2.839-846.1983. [DOI] [PMC free article] [PubMed] [Google Scholar]
- Valentine C. R. One-kilobase direct repeats of plasmid pSa. Plasmid. 1985 Sep;14(2):167–170. doi: 10.1016/0147-619x(85)90077-0. [DOI] [PubMed] [Google Scholar]
- Vieira J., Messing J. The pUC plasmids, an M13mp7-derived system for insertion mutagenesis and sequencing with synthetic universal primers. Gene. 1982 Oct;19(3):259–268. doi: 10.1016/0378-1119(82)90015-4. [DOI] [PubMed] [Google Scholar]
- Wren B. W., Mullany P., Clayton C., Tabaqchali S. Nucleotide sequence of a chloramphenicol acetyl transferase gene from Clostridium difficile. Nucleic Acids Res. 1989 Jun 26;17(12):4877–4877. doi: 10.1093/nar/17.12.4877. [DOI] [PMC free article] [PubMed] [Google Scholar]
- Yanisch-Perron C., Vieira J., Messing J. Improved M13 phage cloning vectors and host strains: nucleotide sequences of the M13mp18 and pUC19 vectors. Gene. 1985;33(1):103–119. doi: 10.1016/0378-1119(85)90120-9. [DOI] [PubMed] [Google Scholar]