Abstract
1. Using a solenoid-operated mixing device, time-resolved measurements were made of shortening and accompanying ATP hydrolysis at 20 degrees C by myofibrils prepared from rabbit psoas muscle. 2. The extent of ATP hydrolysis was determined by an improved Malachite Green method for determination of inorganic phosphate (Pi) in the presence of a large excess of ATP. For the measurement of the change in sarcomere length by phase contrast microscopy, shortening was terminated without delay and artifact by a mixture of 0.2 M-acetate (pH 4.6) and 1.25% (v/v) glutaraldehyde. 3. The shortening velocity per half-sarcomere was 10 microns s-1 in 25 mM-KCl for sarcomere lengths above 1.4 microns, and at least 12 microns s-1 in 150 mM-KCl for sarcomere lengths above 1.7 microns. During this rapid shortening, there was no significant ATP turnover by cross-bridges (upper 95% confidence limit: 0.14 mol (mol of myosin head)-1 in 25 mM-KCl; 0.12 mol mol-1 in KCl solutions greater than or equal to 100 mM). 4. When the sarcomeres shortened below 1.7 microns in KCl concentrations greater than 100 mM or below 1.4 microns in 25 mM-KCl, there was a transient acceleration of ATP hydrolysis (delayed ATP hydrolysis), which was then followed by a steady slow hydrolysis. 5. The magnitudes (+/- estimated standard deviation) of delayed ATP hydrolysis by myofibrils of initial sarcomere length 2.4 microns were 0.42 +/- 0.19, 0.31 +/- 0.10 and 0.17 +/- 0.09 mol (mol myosin head)-1 in 25 mM, 100 mM and 150 mM-KCl, respectively. For myofibrils of sarcomere length 2.0 microns, however, it decreased to 0.24 +/- 0.10 mol mol-1 in 25 mM-KCl or to an insignificant level in 150 mM-KCl. 6. These results indicate that most of the ATP hydrolysis products remain bound to cross-bridges during rapid shortening, and that when the force opposing shortening increases, a proportion of cross-bridges rapidly dissociate the products and enter the next ATP cycle, which diminishes with the decrease in shortening distance as well as the increase in ionic strength. Such behaviour of the cross-bridge is probably a manifestation of its energetic and kinetic properties in the state with bound ADP and Pi interacting with actin filaments at zero load and at a transition from zero to non-zero loads.
Full text
PDF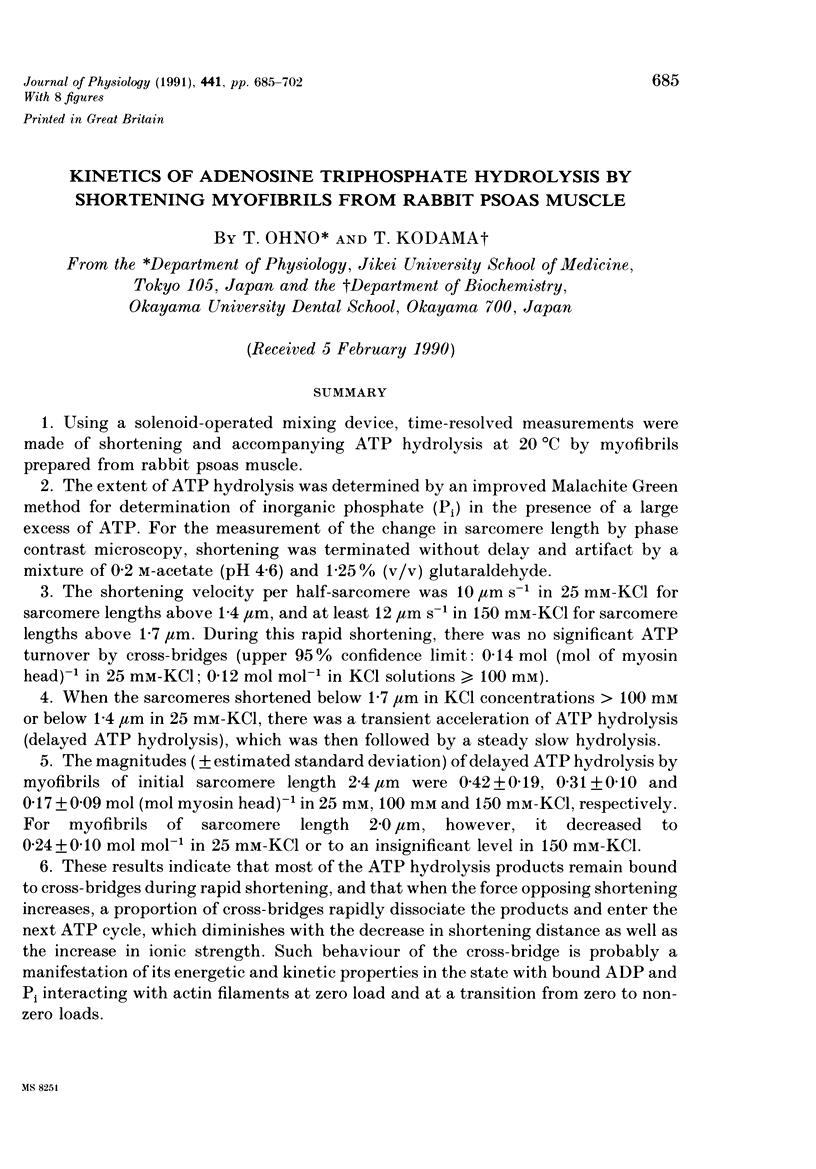
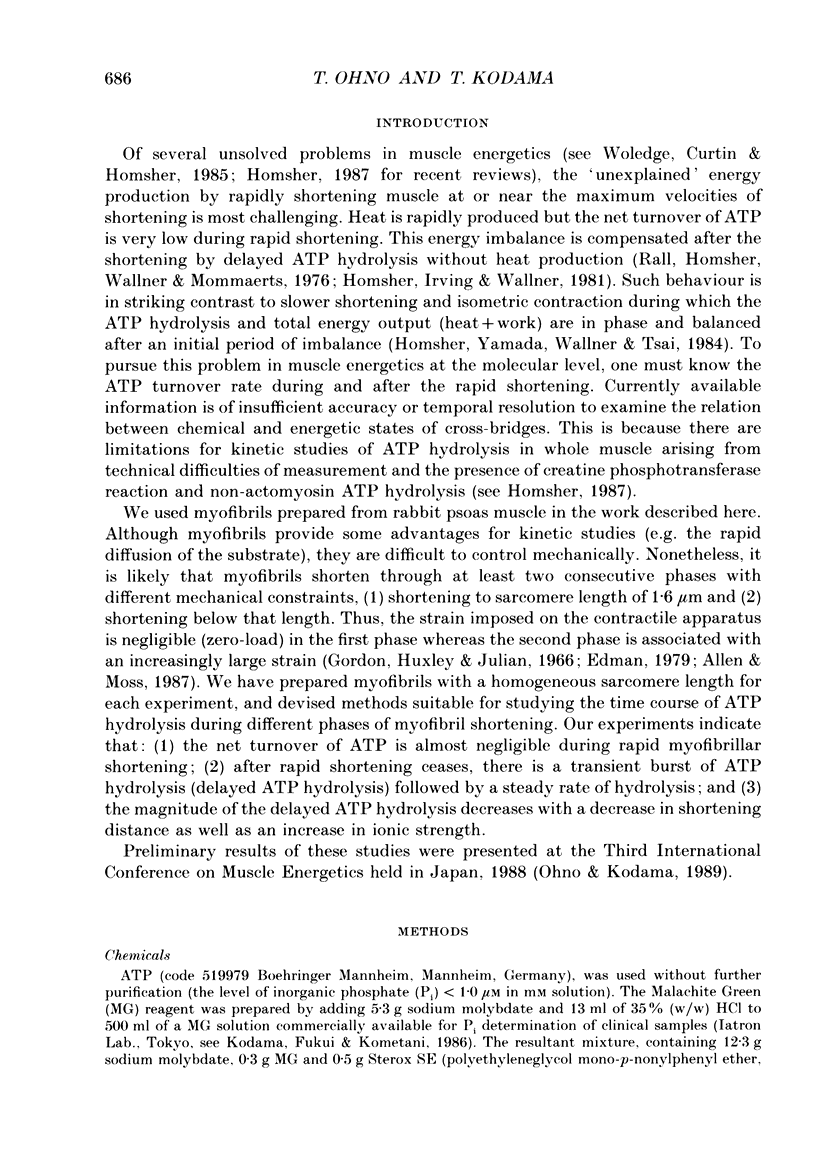
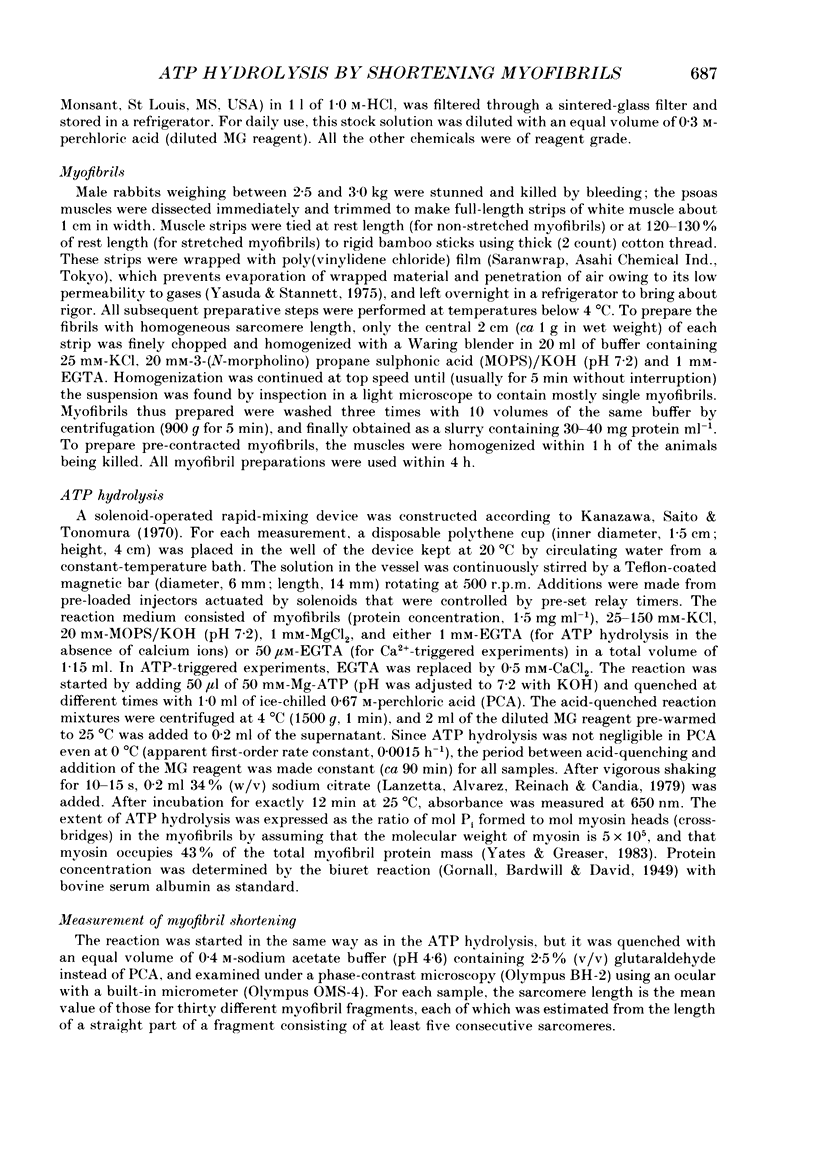
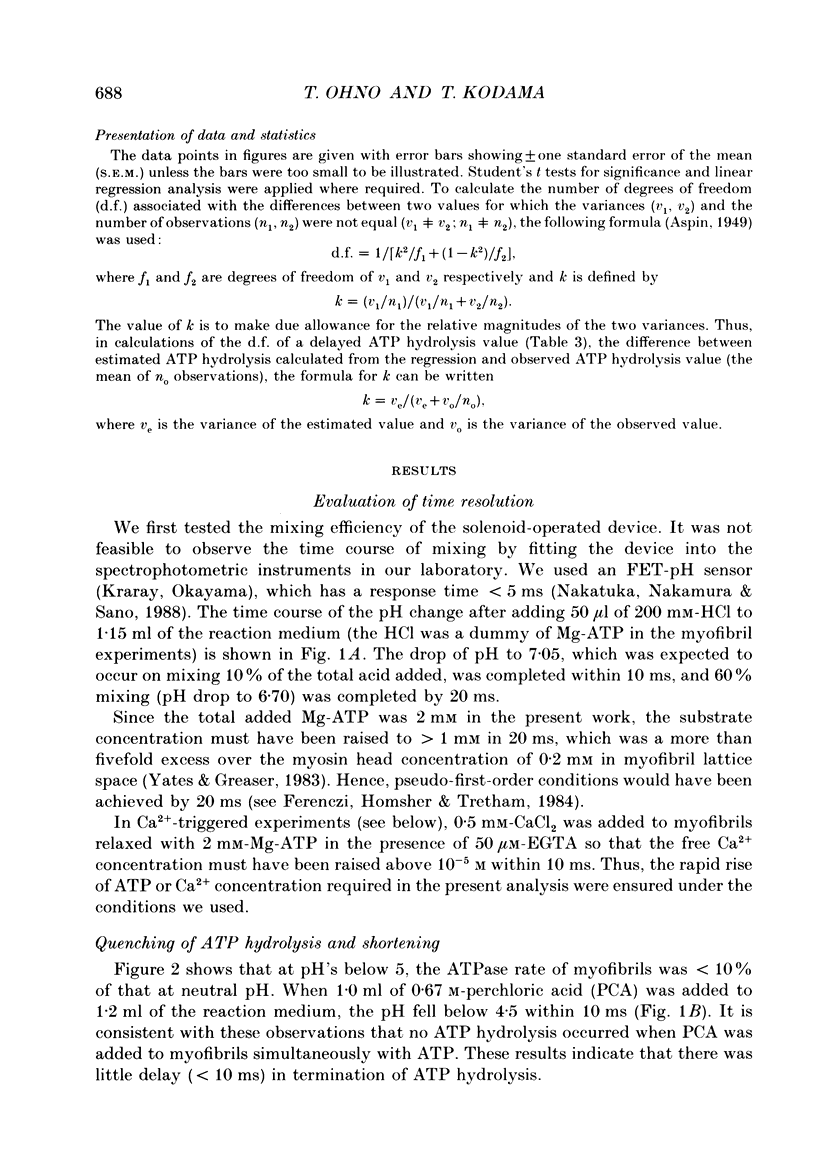
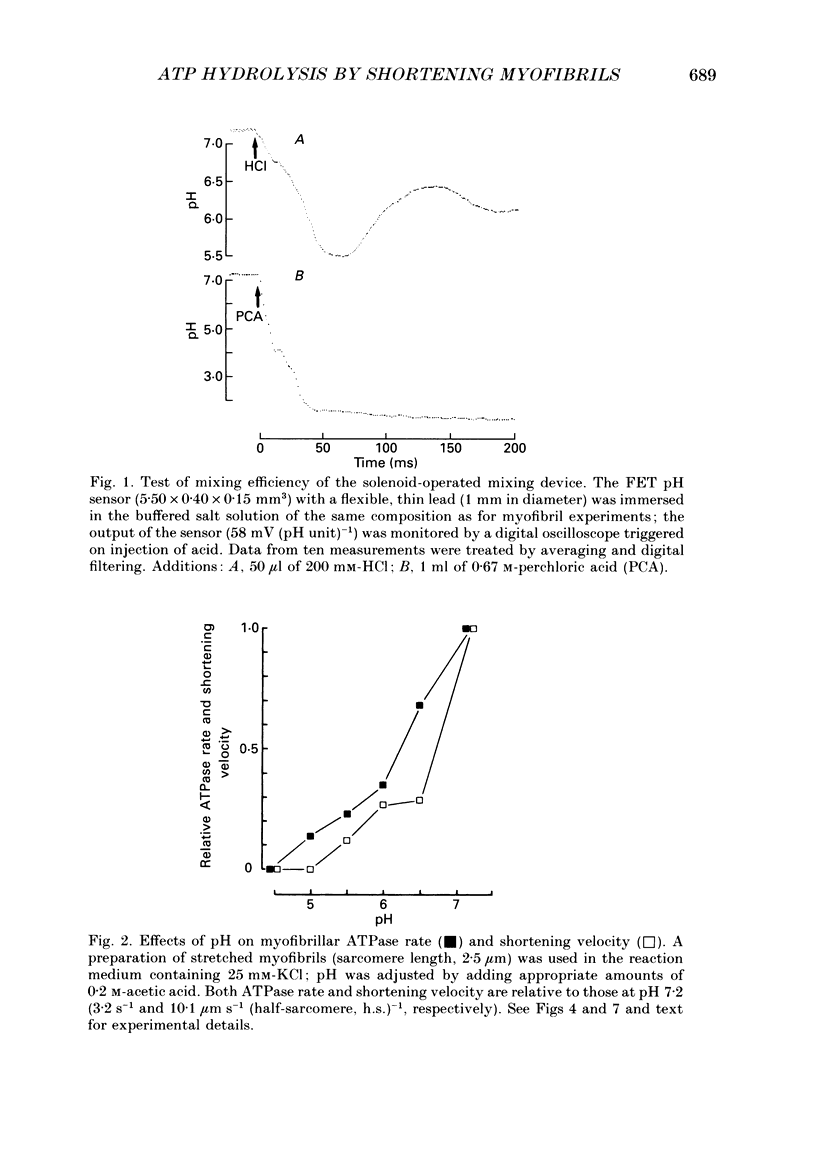
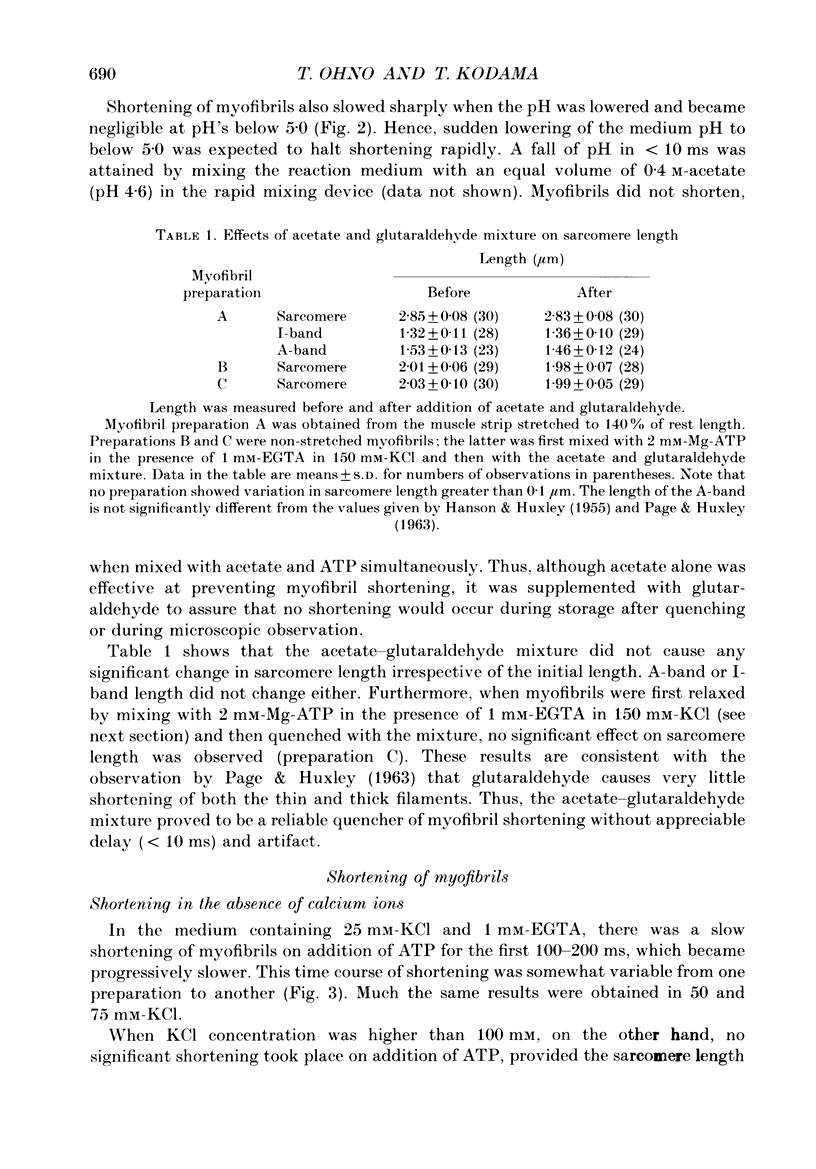
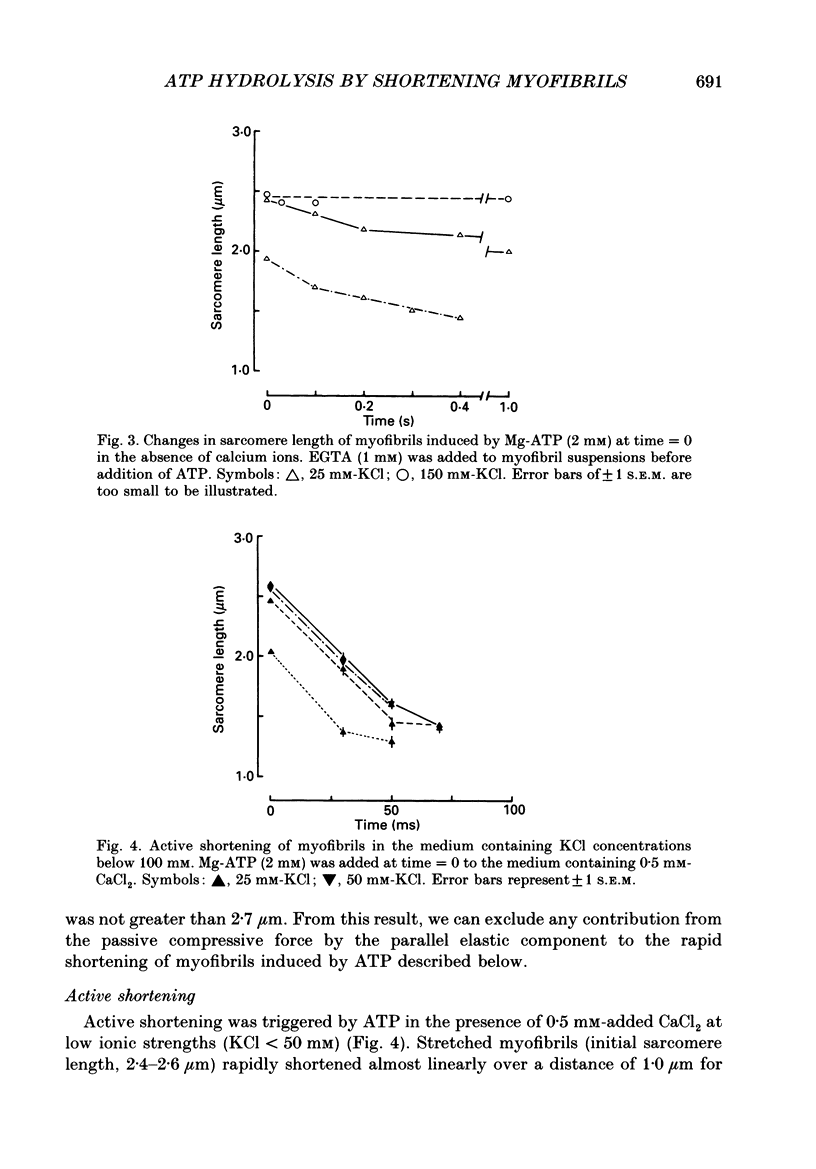
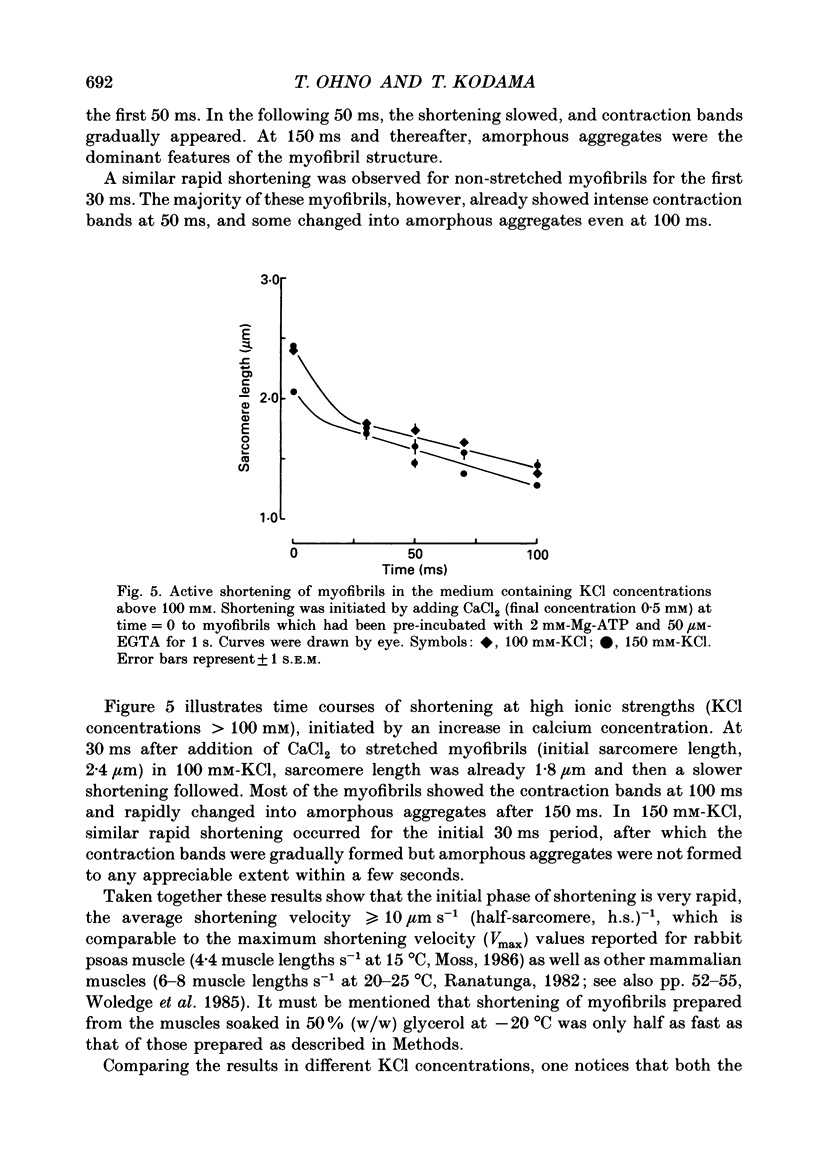
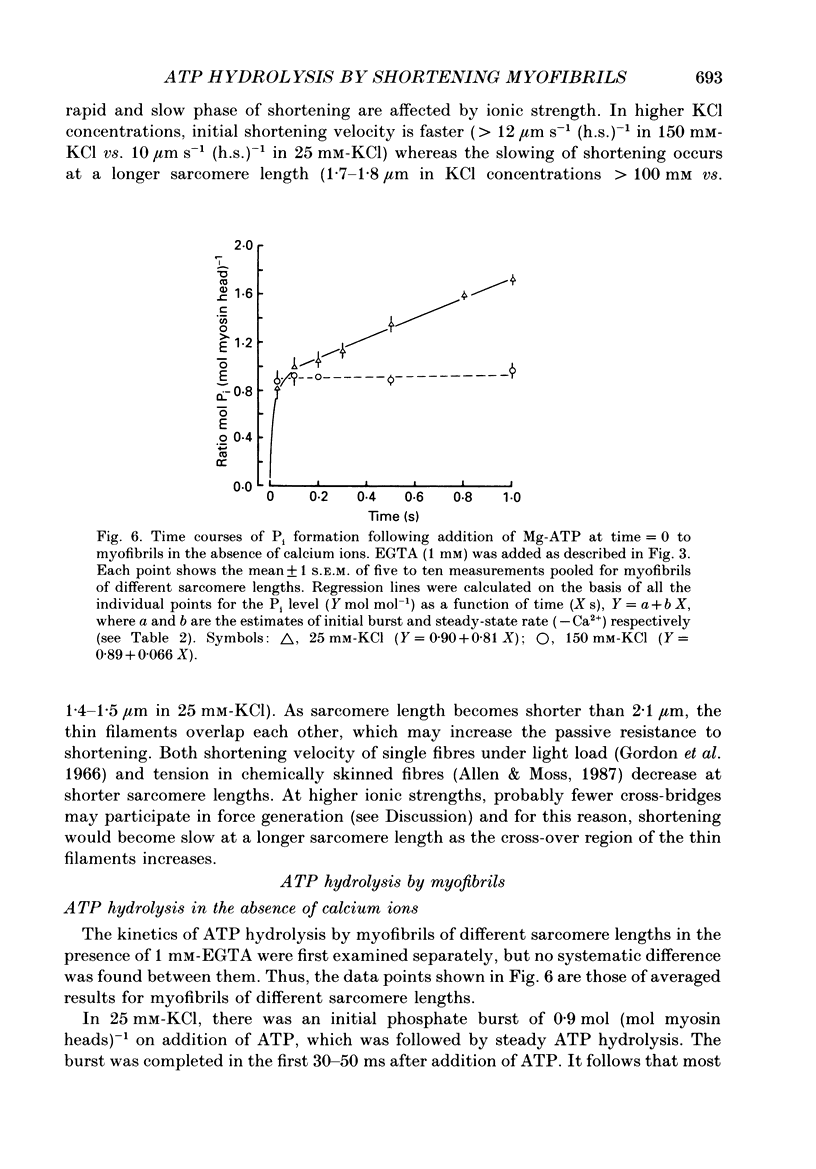
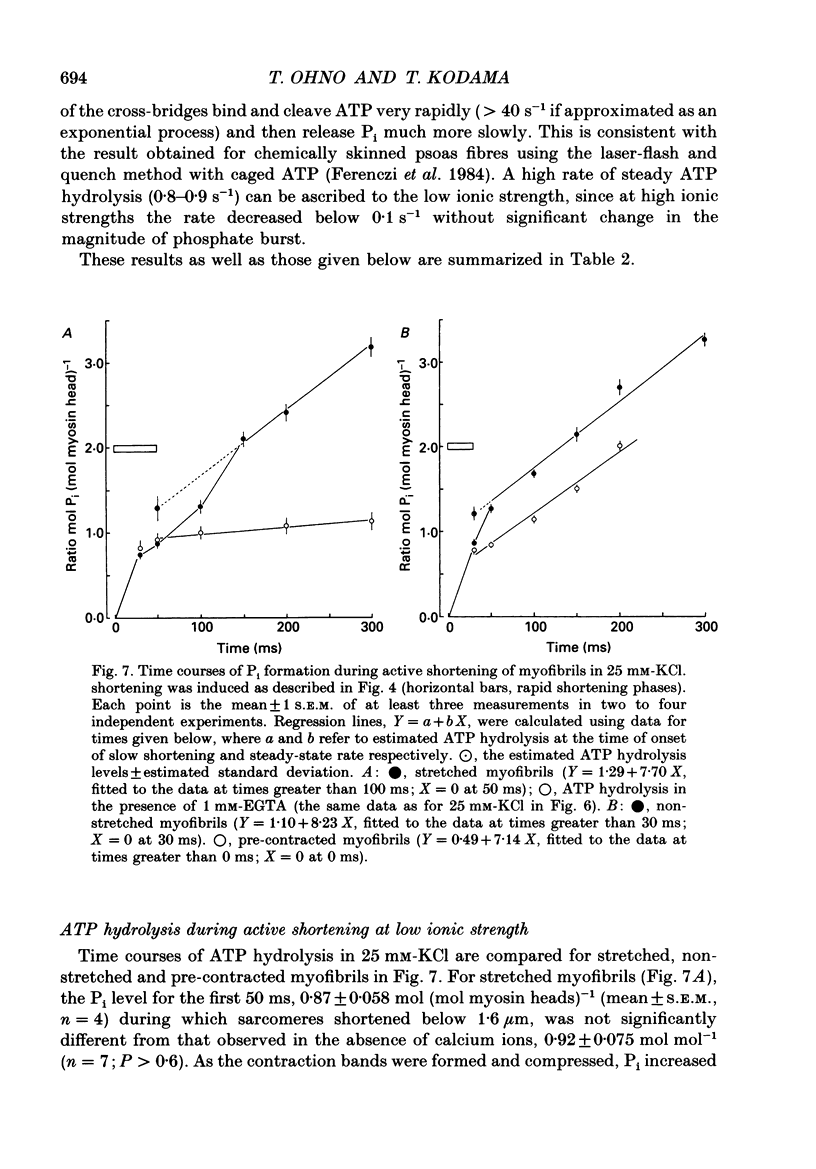
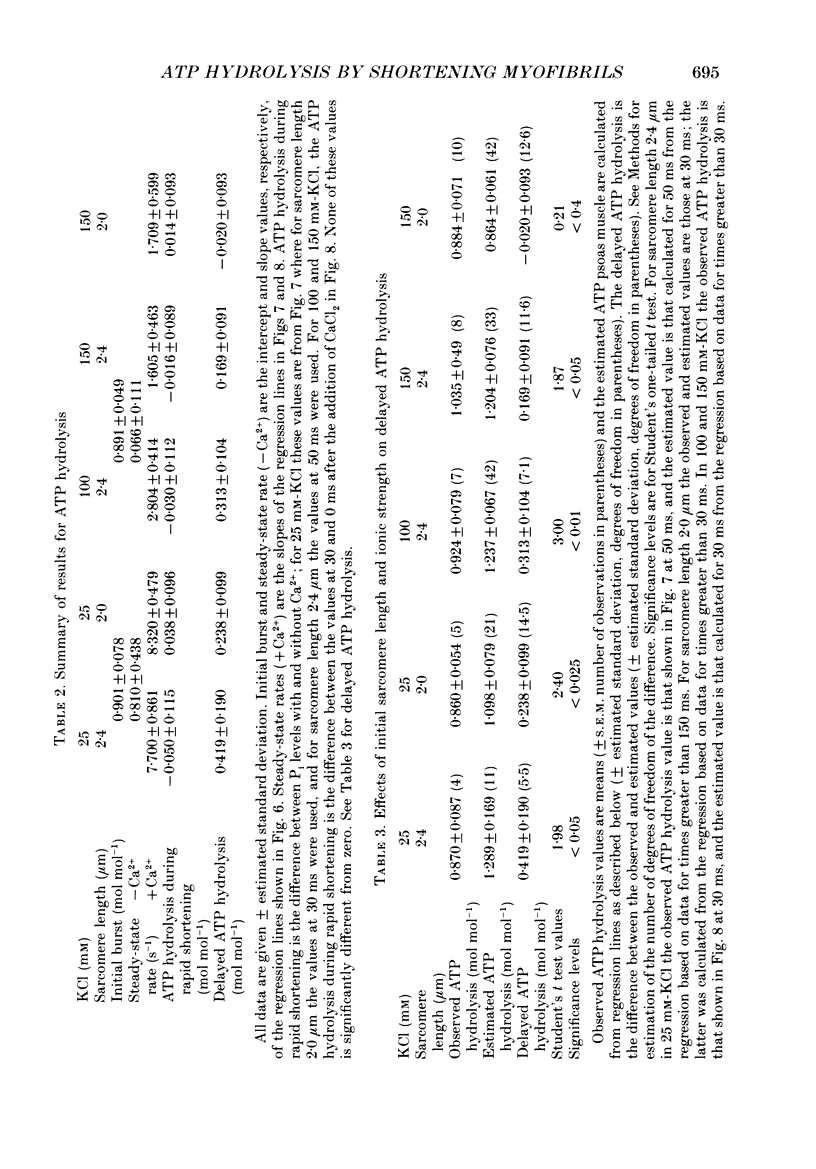
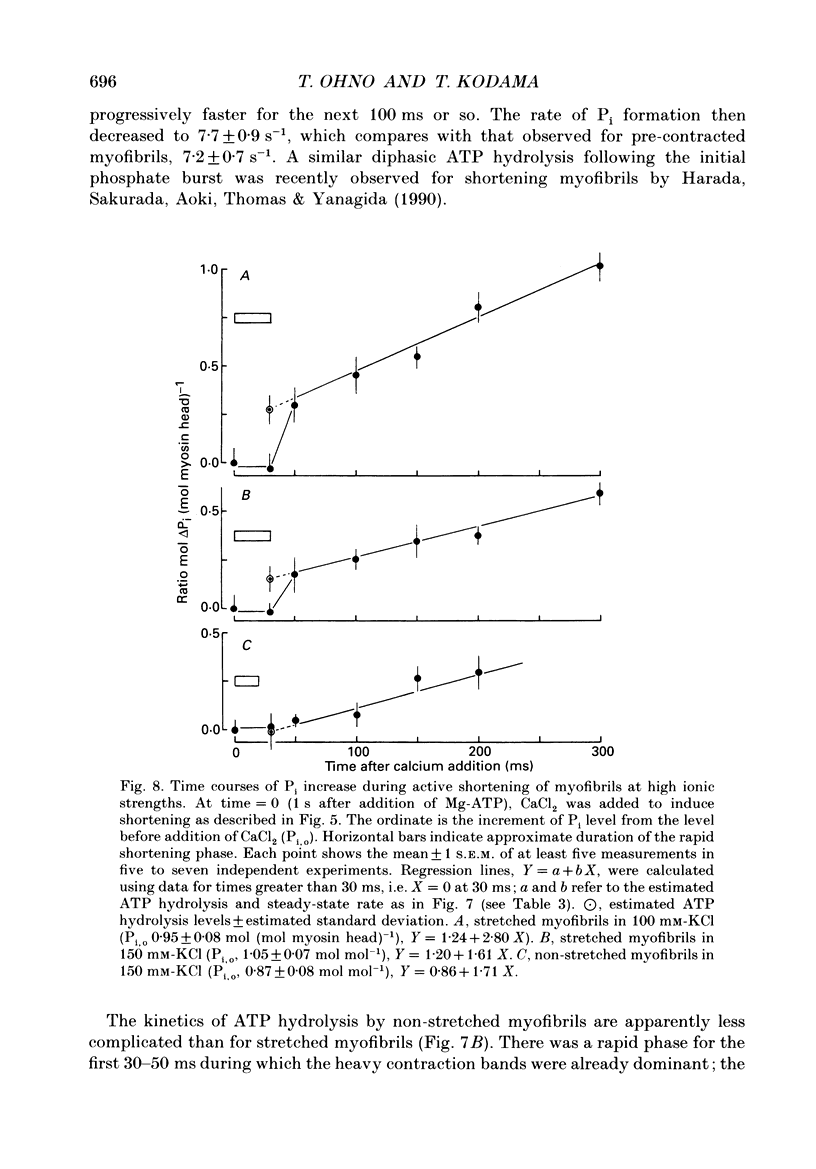
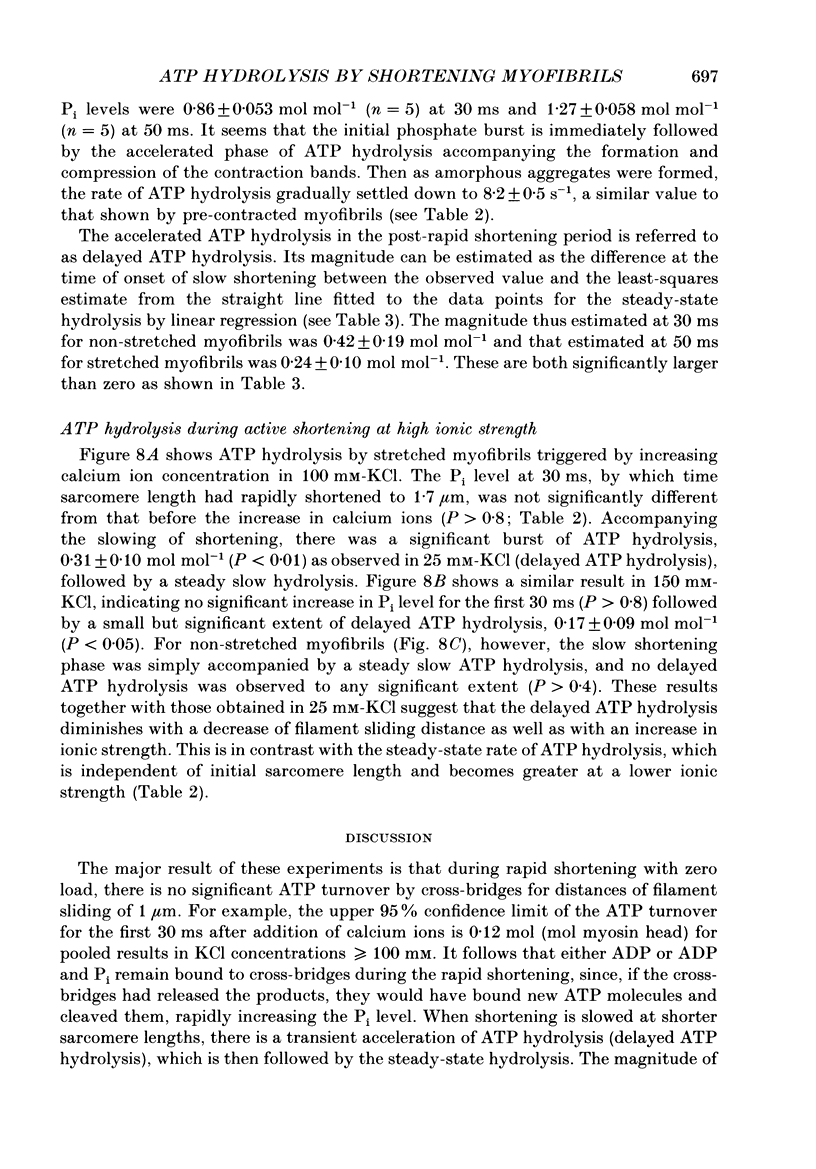
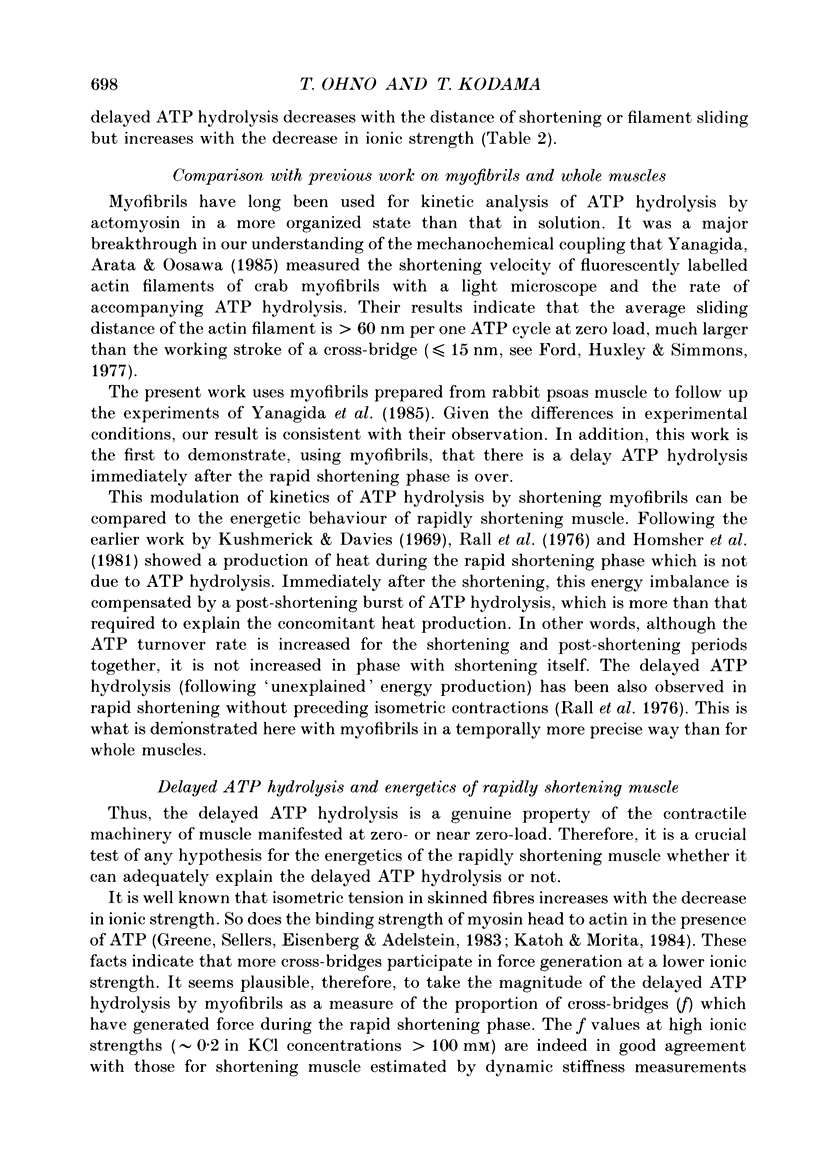
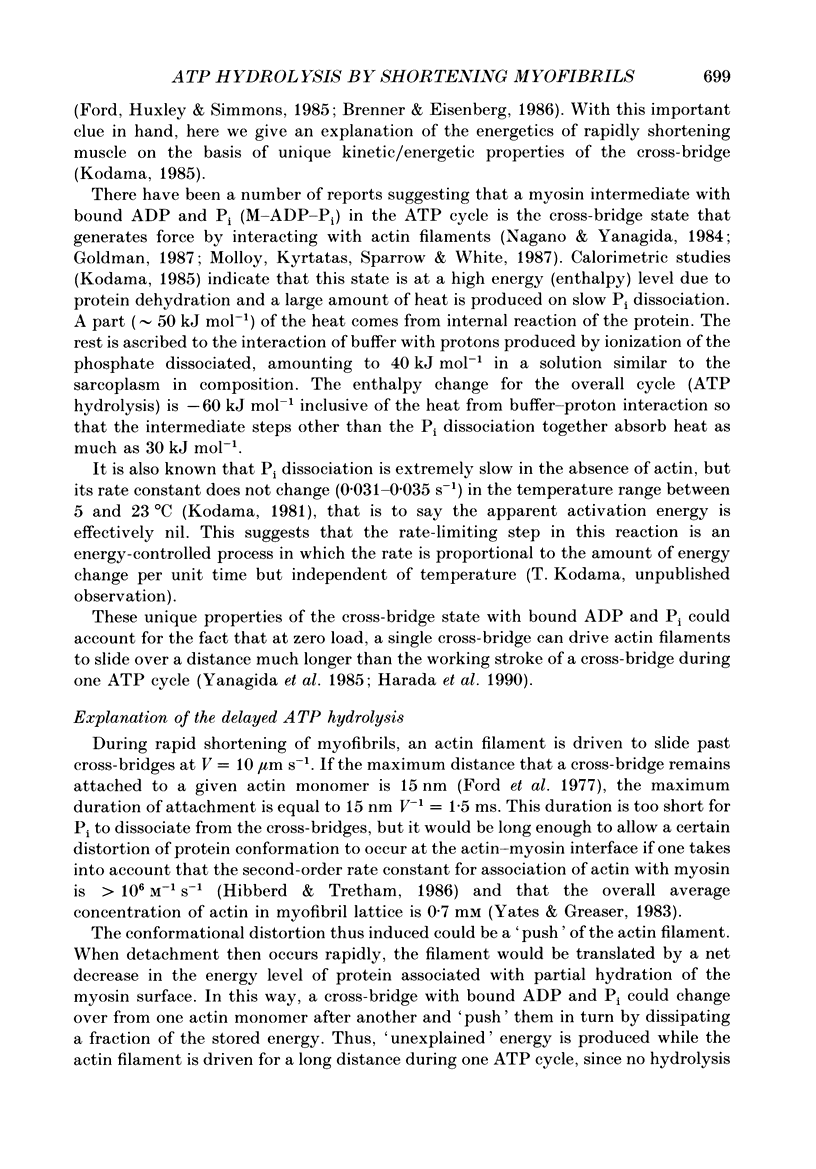
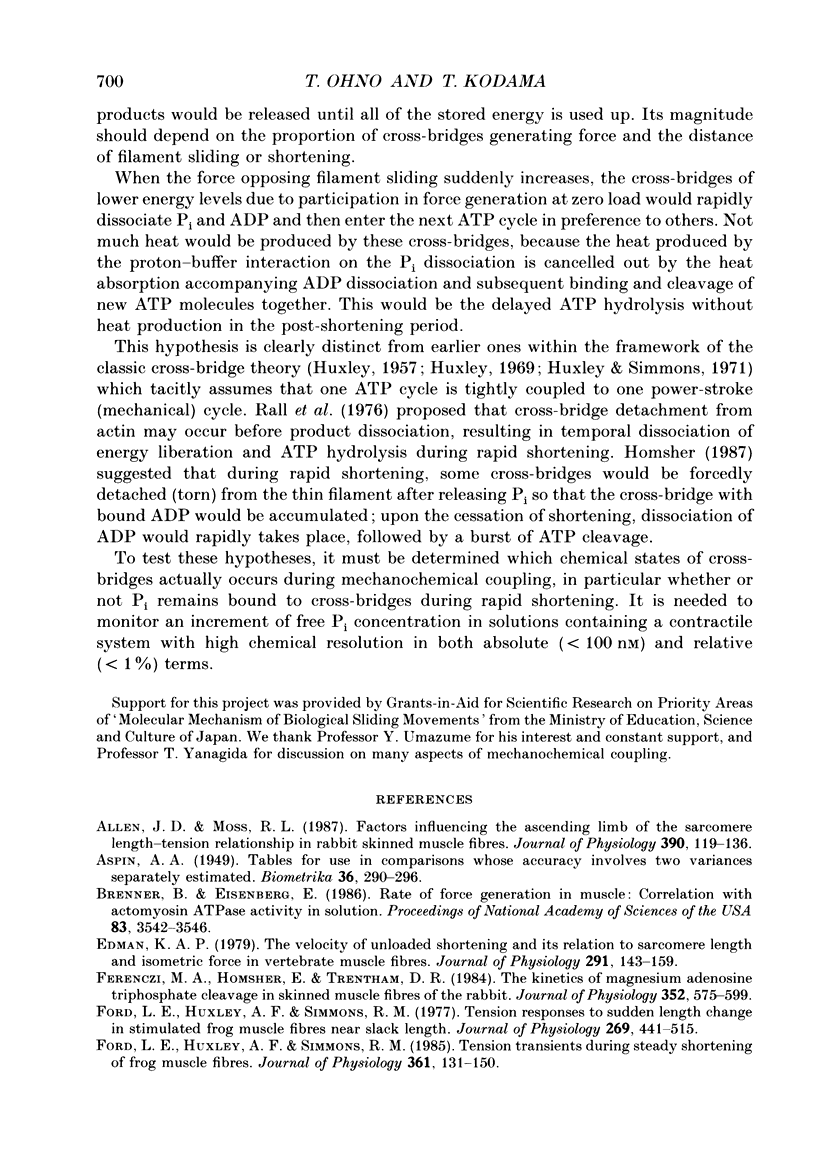
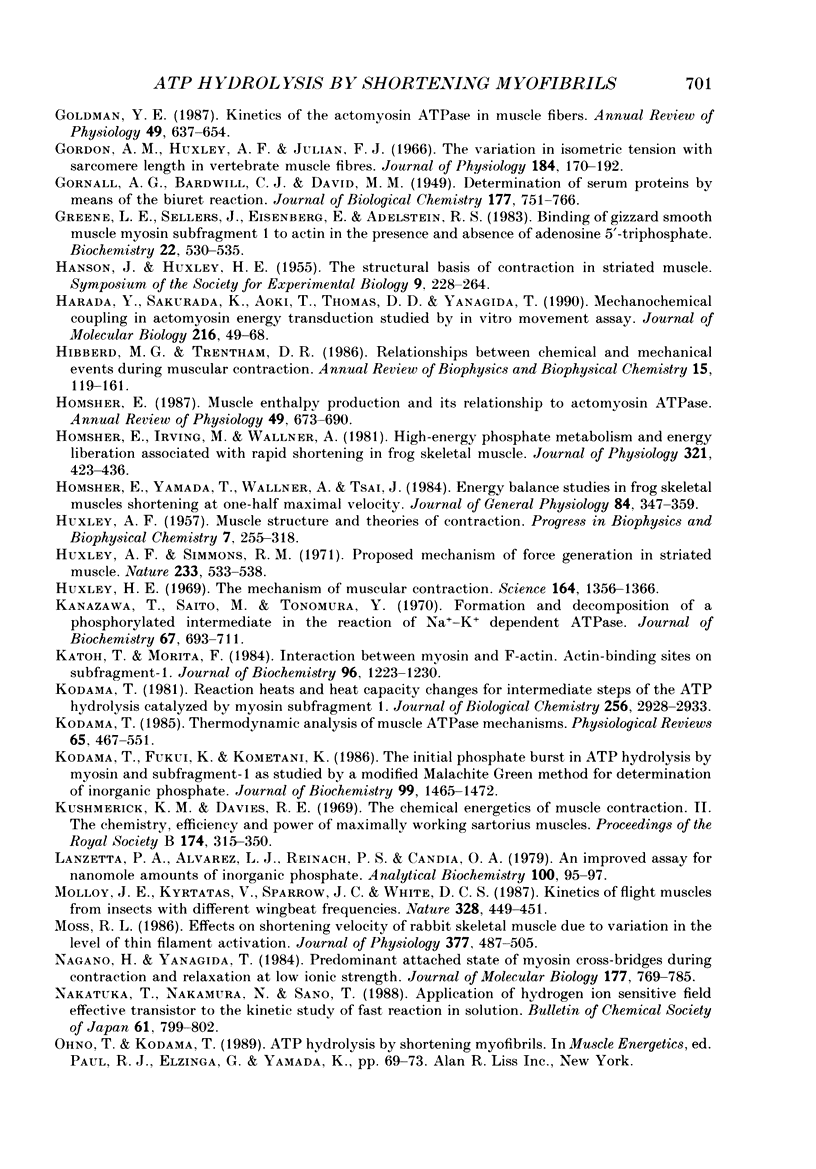
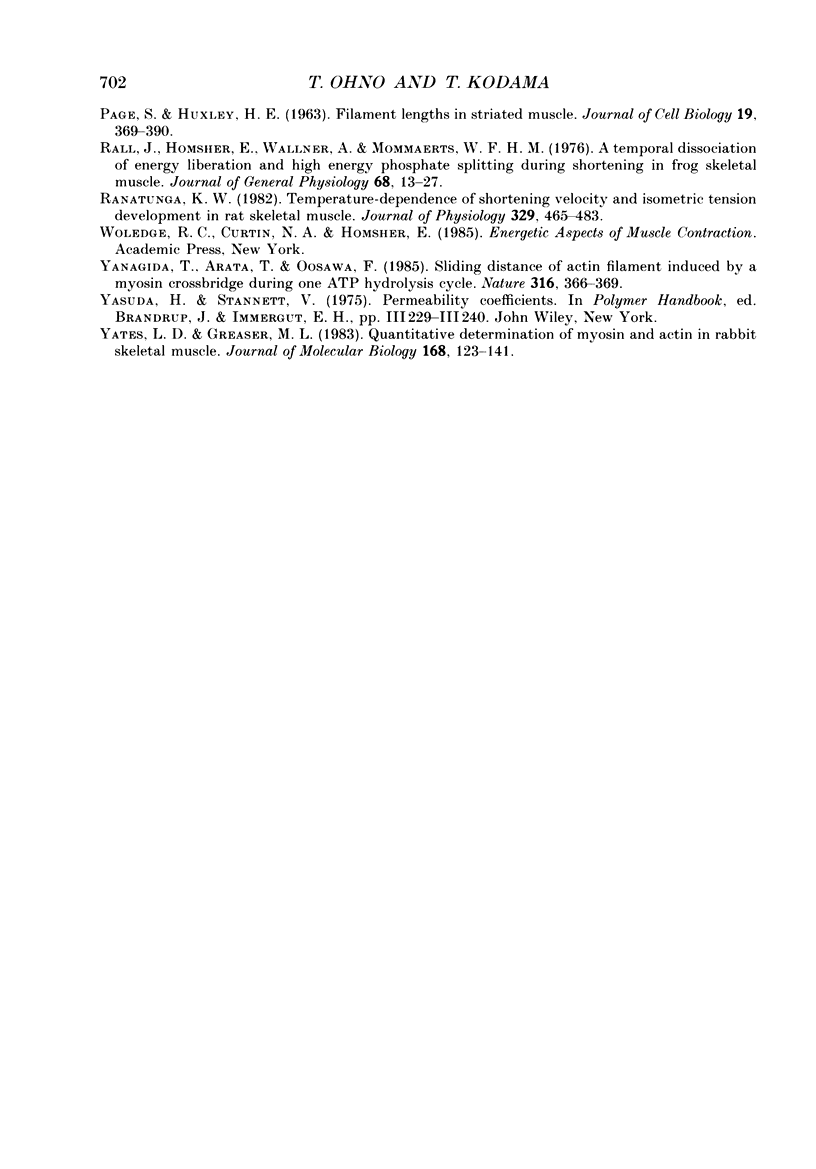
Selected References
These references are in PubMed. This may not be the complete list of references from this article.
- ASPIN A. A. Tables for use in comparisons whose accuracy involves two variances, separately estimated. Biometrika. 1949 Dec;36(3-4):290–296. [PubMed] [Google Scholar]
- Allen J. D., Moss R. L. Factors influencing the ascending limb of the sarcomere length-tension relationship in rabbit skinned muscle fibres. J Physiol. 1987 Sep;390:119–136. doi: 10.1113/jphysiol.1987.sp016689. [DOI] [PMC free article] [PubMed] [Google Scholar]
- Brenner B., Eisenberg E. Rate of force generation in muscle: correlation with actomyosin ATPase activity in solution. Proc Natl Acad Sci U S A. 1986 May;83(10):3542–3546. doi: 10.1073/pnas.83.10.3542. [DOI] [PMC free article] [PubMed] [Google Scholar]
- Edman K. A. The velocity of unloaded shortening and its relation to sarcomere length and isometric force in vertebrate muscle fibres. J Physiol. 1979 Jun;291:143–159. doi: 10.1113/jphysiol.1979.sp012804. [DOI] [PMC free article] [PubMed] [Google Scholar]
- Ferenczi M. A., Homsher E., Trentham D. R. The kinetics of magnesium adenosine triphosphate cleavage in skinned muscle fibres of the rabbit. J Physiol. 1984 Jul;352:575–599. doi: 10.1113/jphysiol.1984.sp015311. [DOI] [PMC free article] [PubMed] [Google Scholar]
- Ford L. E., Huxley A. F., Simmons R. M. Tension responses to sudden length change in stimulated frog muscle fibres near slack length. J Physiol. 1977 Jul;269(2):441–515. doi: 10.1113/jphysiol.1977.sp011911. [DOI] [PMC free article] [PubMed] [Google Scholar]
- Ford L. E., Huxley A. F., Simmons R. M. Tension transients during steady shortening of frog muscle fibres. J Physiol. 1985 Apr;361:131–150. doi: 10.1113/jphysiol.1985.sp015637. [DOI] [PMC free article] [PubMed] [Google Scholar]
- GORNALL A. G., BARDAWILL C. J., DAVID M. M. Determination of serum proteins by means of the biuret reaction. J Biol Chem. 1949 Feb;177(2):751–766. [PubMed] [Google Scholar]
- Goldman Y. E. Kinetics of the actomyosin ATPase in muscle fibers. Annu Rev Physiol. 1987;49:637–654. doi: 10.1146/annurev.ph.49.030187.003225. [DOI] [PubMed] [Google Scholar]
- Gordon A. M., Huxley A. F., Julian F. J. The variation in isometric tension with sarcomere length in vertebrate muscle fibres. J Physiol. 1966 May;184(1):170–192. doi: 10.1113/jphysiol.1966.sp007909. [DOI] [PMC free article] [PubMed] [Google Scholar]
- Greene L. E., Sellers J. R., Eisenberg E., Adelstein R. S. Binding of gizzard smooth muscle myosin subfragment 1 to actin in the presence and absence of adenosine 5'-triphosphate. Biochemistry. 1983 Feb 1;22(3):530–535. doi: 10.1021/bi00272a002. [DOI] [PubMed] [Google Scholar]
- HUXLEY A. F. Muscle structure and theories of contraction. Prog Biophys Biophys Chem. 1957;7:255–318. [PubMed] [Google Scholar]
- Harada Y., Sakurada K., Aoki T., Thomas D. D., Yanagida T. Mechanochemical coupling in actomyosin energy transduction studied by in vitro movement assay. J Mol Biol. 1990 Nov 5;216(1):49–68. doi: 10.1016/S0022-2836(05)80060-9. [DOI] [PubMed] [Google Scholar]
- Hibberd M. G., Trentham D. R. Relationships between chemical and mechanical events during muscular contraction. Annu Rev Biophys Biophys Chem. 1986;15:119–161. doi: 10.1146/annurev.bb.15.060186.001003. [DOI] [PubMed] [Google Scholar]
- Homsher E., Irving M., Wallner A. High-energy phosphate metabolism and energy liberation associated with rapid shortening in frog skeletal muscle. J Physiol. 1981 Dec;321:423–436. doi: 10.1113/jphysiol.1981.sp013994. [DOI] [PMC free article] [PubMed] [Google Scholar]
- Homsher E. Muscle enthalpy production and its relationship to actomyosin ATPase. Annu Rev Physiol. 1987;49:673–690. doi: 10.1146/annurev.ph.49.030187.003325. [DOI] [PubMed] [Google Scholar]
- Homsher E., Yamada T., Wallner A., Tsai J. Energy balance studies in frog skeletal muscles shortening at one-half maximal velocity. J Gen Physiol. 1984 Sep;84(3):347–359. doi: 10.1085/jgp.84.3.347. [DOI] [PMC free article] [PubMed] [Google Scholar]
- Huxley A. F., Simmons R. M. Proposed mechanism of force generation in striated muscle. Nature. 1971 Oct 22;233(5321):533–538. doi: 10.1038/233533a0. [DOI] [PubMed] [Google Scholar]
- Huxley H. E. The mechanism of muscular contraction. Science. 1969 Jun 20;164(3886):1356–1365. doi: 10.1126/science.164.3886.1356. [DOI] [PubMed] [Google Scholar]
- Kanazawa T., Saito M., Tonomura Y. Formation and decomposition of a phosphorylated intermediate in the reaction of Na plus-K plus dependent ATPase. J Biochem. 1970 May;67(5):693–711. doi: 10.1093/oxfordjournals.jbchem.a129297. [DOI] [PubMed] [Google Scholar]
- Katoh T., Morita F. Interaction between myosin and F-actin. Correlation with actin-binding sites on subfragment-1. J Biochem. 1984 Oct;96(4):1223–1230. doi: 10.1093/oxfordjournals.jbchem.a134940. [DOI] [PubMed] [Google Scholar]
- Kodama T., Fukui K., Kometani K. The initial phosphate burst in ATP hydrolysis by myosin and subfragment-1 as studied by a modified malachite green method for determination of inorganic phosphate. J Biochem. 1986 May;99(5):1465–1472. doi: 10.1093/oxfordjournals.jbchem.a135616. [DOI] [PubMed] [Google Scholar]
- Kodama T. Reaction heats and heat capacity changes for intermediate steps of the ATP hydrolysis catalyzed by myosin subfragment 1. J Biol Chem. 1981 Mar 25;256(6):2928–2933. [PubMed] [Google Scholar]
- Kodama T. Thermodynamic analysis of muscle ATPase mechanisms. Physiol Rev. 1985 Apr;65(2):467–551. doi: 10.1152/physrev.1985.65.2.467. [DOI] [PubMed] [Google Scholar]
- Kushmerick M. J., Davies R. E. The chemical energetics of muscle contraction. II. The chemistry, efficiency and power of maximally working sartorius muscles. Appendix. Free energy and enthalpy of atp hydrolysis in the sarcoplasm. Proc R Soc Lond B Biol Sci. 1969 Dec 23;174(1036):315–353. doi: 10.1098/rspb.1969.0096. [DOI] [PubMed] [Google Scholar]
- Lanzetta P. A., Alvarez L. J., Reinach P. S., Candia O. A. An improved assay for nanomole amounts of inorganic phosphate. Anal Biochem. 1979 Nov 15;100(1):95–97. doi: 10.1016/0003-2697(79)90115-5. [DOI] [PubMed] [Google Scholar]
- Moss R. L. Effects on shortening velocity of rabbit skeletal muscle due to variations in the level of thin-filament activation. J Physiol. 1986 Aug;377:487–505. doi: 10.1113/jphysiol.1986.sp016199. [DOI] [PMC free article] [PubMed] [Google Scholar]
- Nagano H., Yanagida T. Predominant attached state of myosin cross-bridges during contraction and relaxation at low ionic strength. J Mol Biol. 1984 Aug 25;177(4):769–785. doi: 10.1016/0022-2836(84)90048-2. [DOI] [PubMed] [Google Scholar]
- Ohno T., Kodama T. ATP hydrolysis by shortening myofibrils. Prog Clin Biol Res. 1989;315:69–73. [PubMed] [Google Scholar]
- PAGE S. G., HUXLEY H. E. FILAMENT LENGTHS IN STRIATED MUSCLE. J Cell Biol. 1963 Nov;19:369–390. doi: 10.1083/jcb.19.2.369. [DOI] [PMC free article] [PubMed] [Google Scholar]
- Rall J. A., Homsher E., Wallner A., Mommaerts W. F. A temporal dissociation of energy liberation and high energy phosphate splitting during shortening in frog skeletal muscles. J Gen Physiol. 1976 Jul;68(1):13–27. doi: 10.1085/jgp.68.1.13. [DOI] [PMC free article] [PubMed] [Google Scholar]
- Ranatunga K. W. Temperature-dependence of shortening velocity and rate of isometric tension development in rat skeletal muscle. J Physiol. 1982 Aug;329:465–483. doi: 10.1113/jphysiol.1982.sp014314. [DOI] [PMC free article] [PubMed] [Google Scholar]
- Yanagida T., Arata T., Oosawa F. Sliding distance of actin filament induced by a myosin crossbridge during one ATP hydrolysis cycle. Nature. 1985 Jul 25;316(6026):366–369. doi: 10.1038/316366a0. [DOI] [PubMed] [Google Scholar]
- Yates L. D., Greaser M. L. Quantitative determination of myosin and actin in rabbit skeletal muscle. J Mol Biol. 1983 Jul 25;168(1):123–141. doi: 10.1016/s0022-2836(83)80326-x. [DOI] [PubMed] [Google Scholar]