Abstract
Model compound studies in the literature show how Hofmeister ion interactions affect protein stability. Although model compound results are typically obtained as salting-out constants, they can be used to find out how the interactions affect protein stability. The null point in the Hofmeister series, which divides protein denaturants from stabilizers, arises from opposite interactions with different classes of groups: Hofmeister ions salt out nonpolar groups and salt in the peptide group. Theories of how Hofmeister ion interactions work need to begin by explaining the mechanisms of these two classes of interactions. Salting-out nonpolar groups has been explained by the cavity model, but its use is controversial. When applied to model compound data, the cavity model 1) uses surface tension increments to predict the observed values of the salting-out constants, within a factor of 3, and 2) predicts that the salting-out constant should increase with the number of carbon atoms in the aliphatic side chain of an amino acid, as observed. The mechanism of interaction between Hofmeister ions and the peptide group is not well understood, and it is controversial whether this interaction is ion-specific, or whether it is nonspecific and the apparent specificity resides in interactions with nearby nonpolar groups. A nonspecific salting-in interaction is known to occur between simple ions and dipolar molecules; it depends on ionic strength, not on position in the Hofmeister series. A theory by Kirkwood predicts the strength of this interaction and indicates that it depends on the first power of the ionic strength. Ions interact with proteins in various ways besides the Hofmeister ion interactions discussed here, especially by charge interactions. Much of what is known about these interactions comes from studies by Serge Timasheff and his co-workers. A general model, suitable for analyzing diverse ion-protein interactions, is provided by the two-domain model of Record and co-workers.
Full text
PDF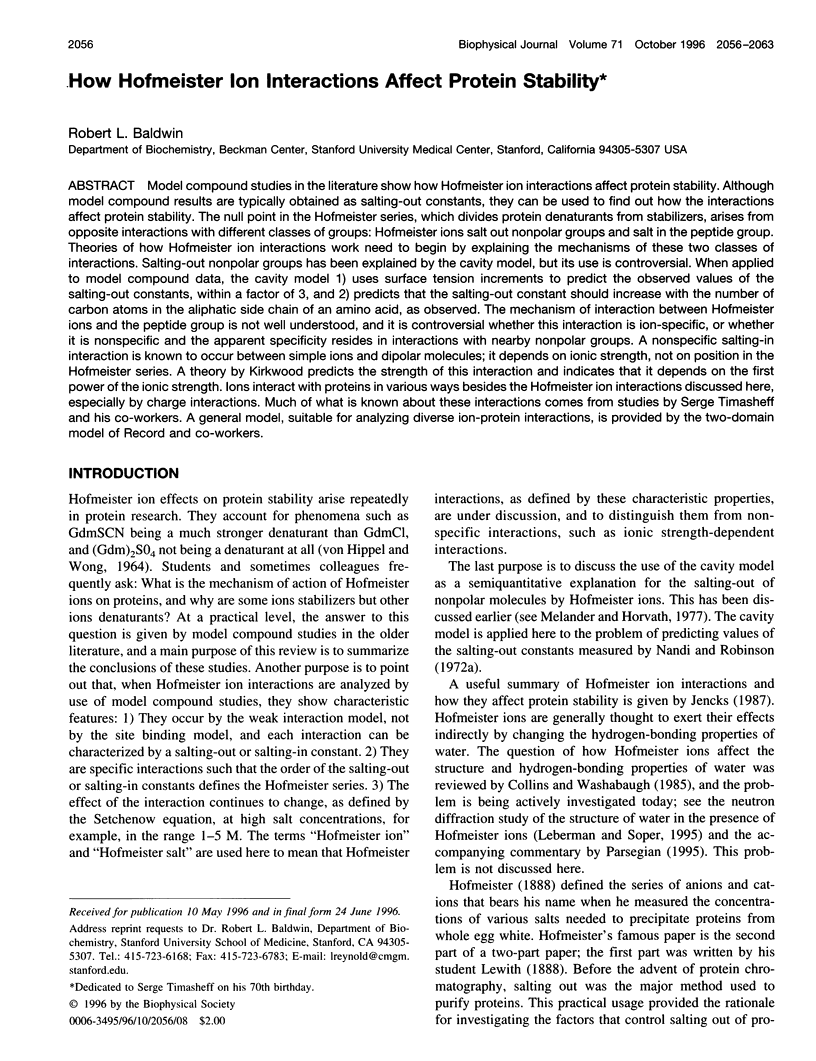
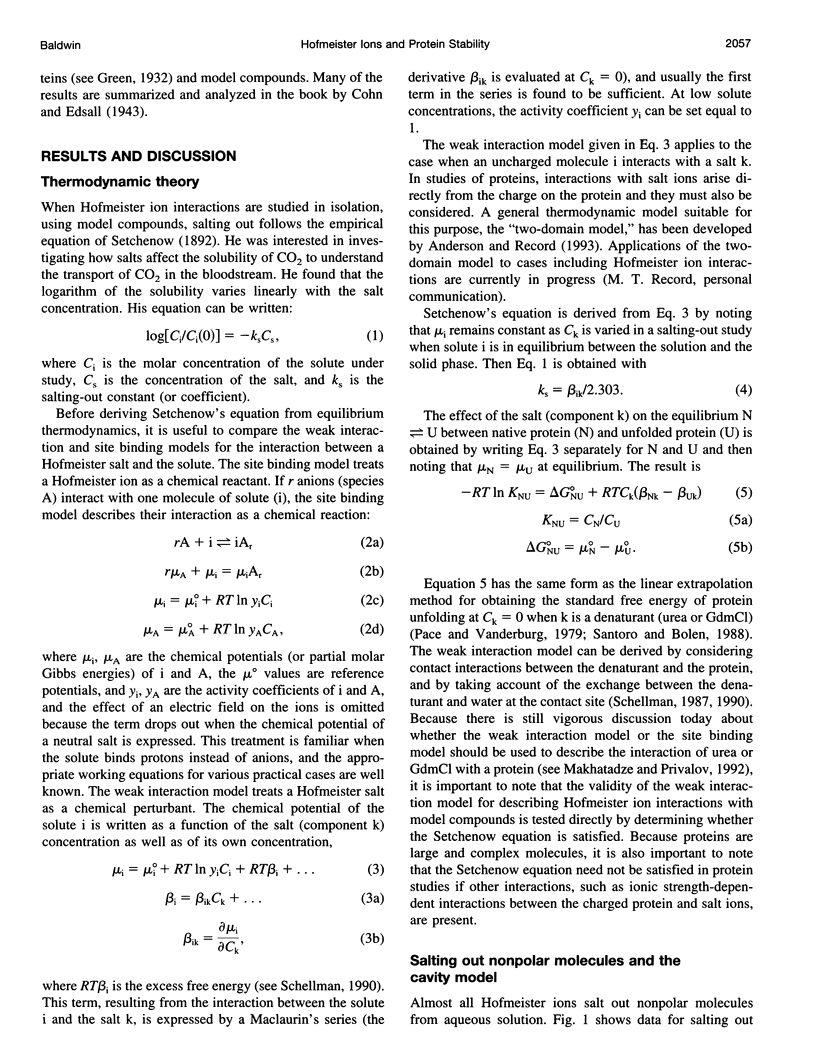
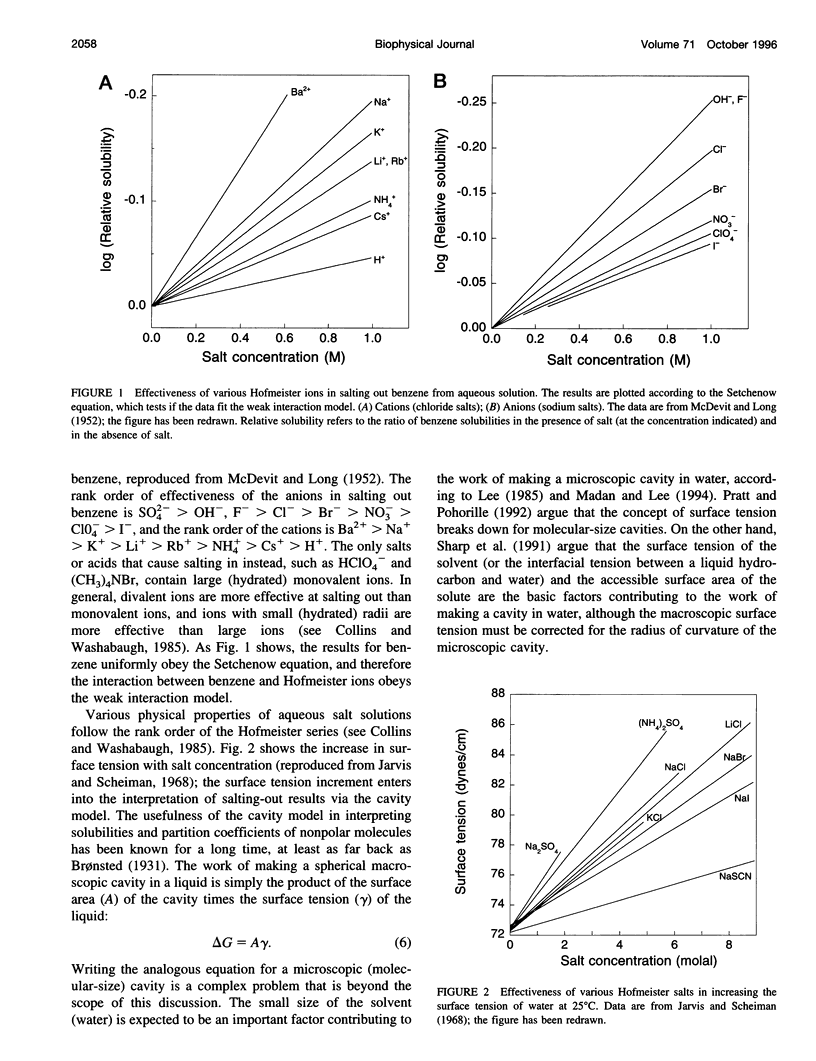
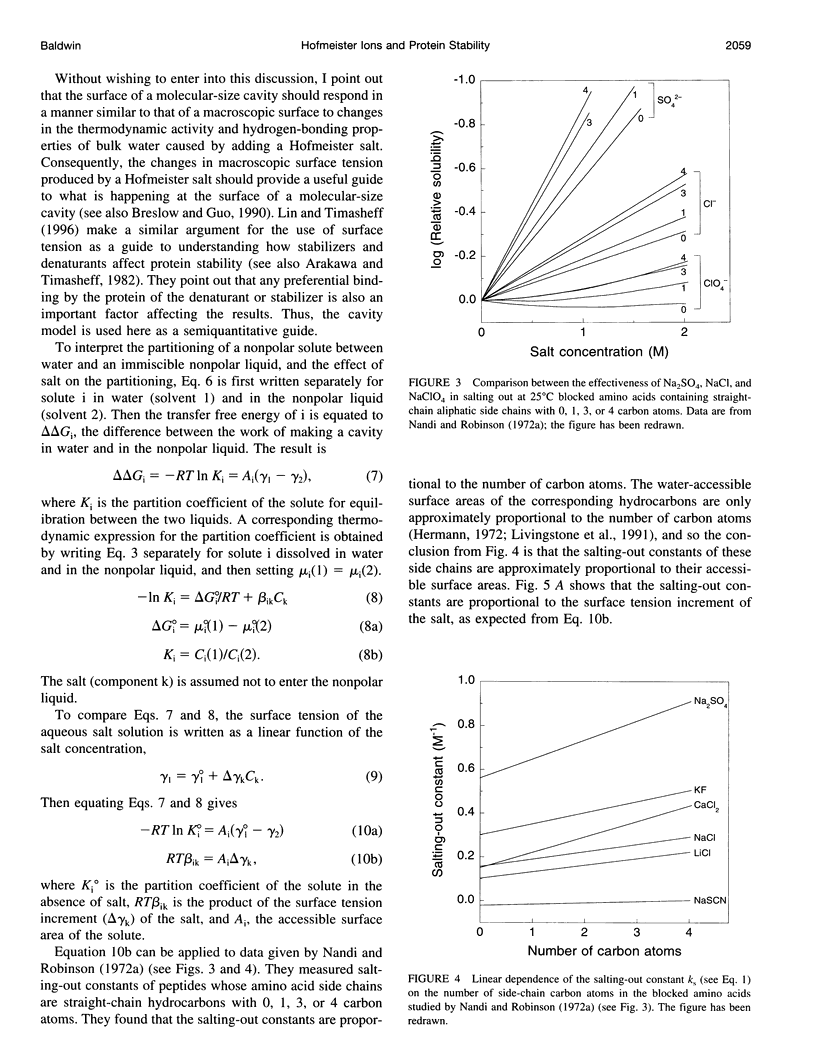
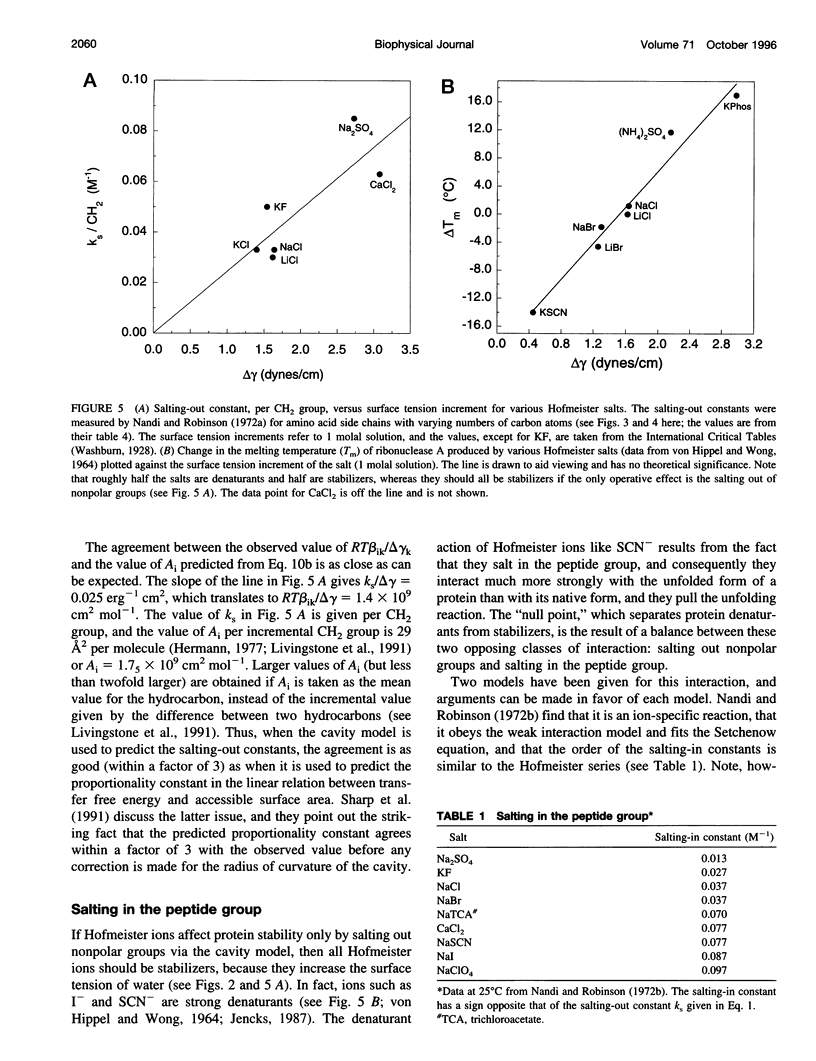
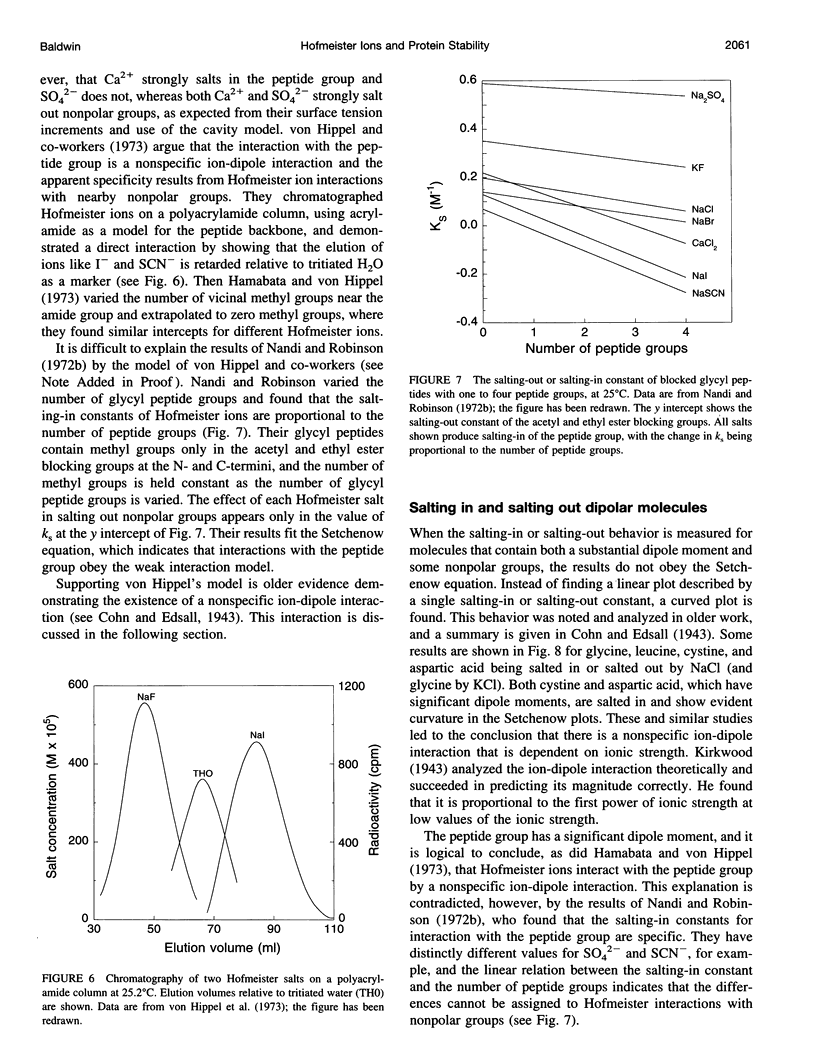
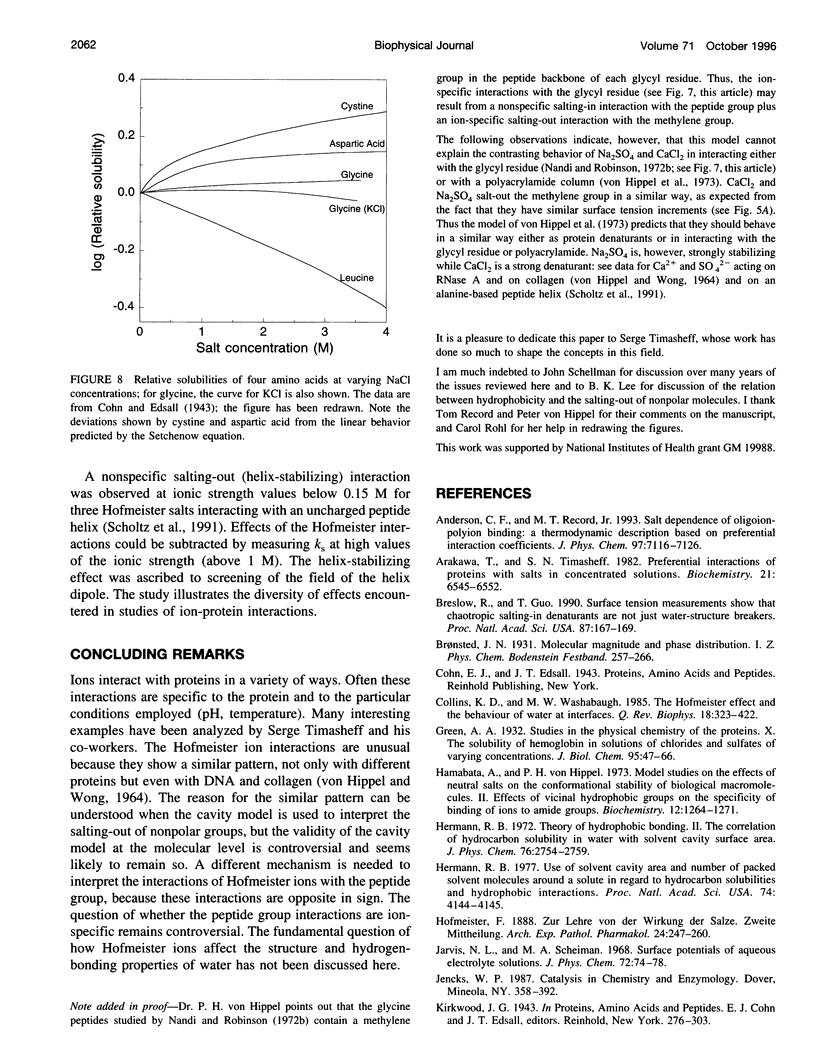
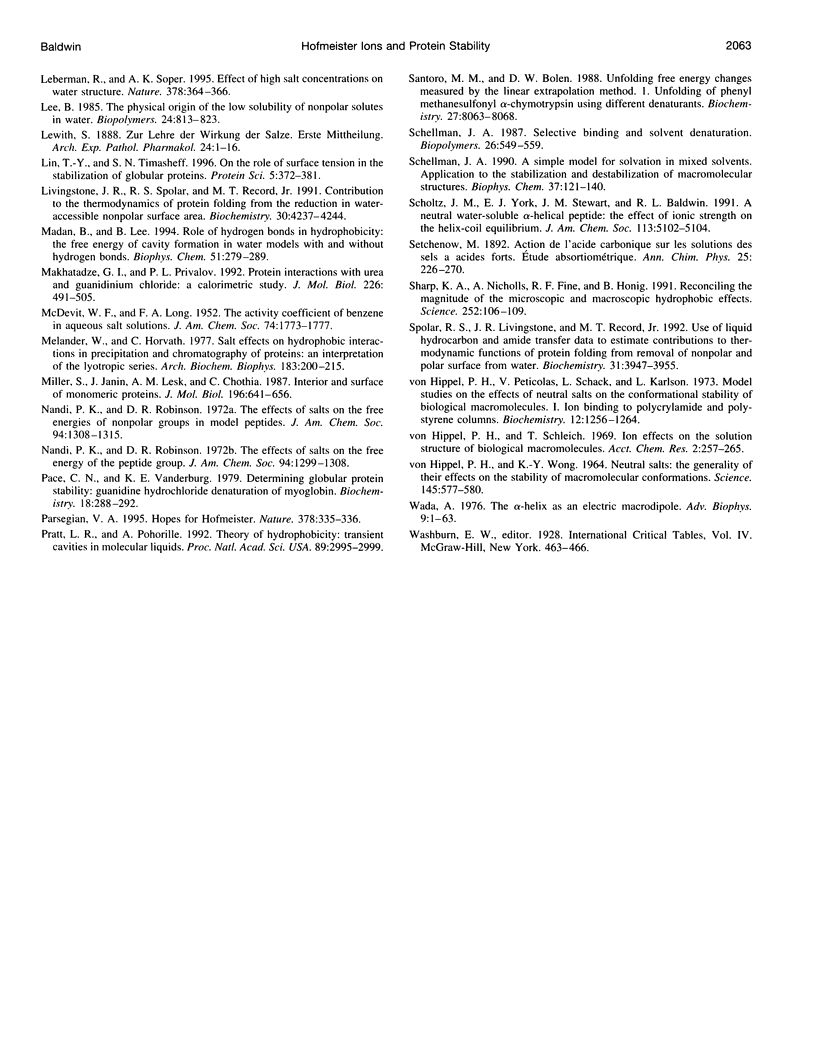
Selected References
These references are in PubMed. This may not be the complete list of references from this article.
- Arakawa T., Timasheff S. N. Preferential interactions of proteins with salts in concentrated solutions. Biochemistry. 1982 Dec 7;21(25):6545–6552. doi: 10.1021/bi00268a034. [DOI] [PubMed] [Google Scholar]
- Breslow R., Guo T. Surface tension measurements show that chaotropic salting-in denaturants are not just water-structure breakers. Proc Natl Acad Sci U S A. 1990 Jan;87(1):167–169. doi: 10.1073/pnas.87.1.167. [DOI] [PMC free article] [PubMed] [Google Scholar]
- Collins K. D., Washabaugh M. W. The Hofmeister effect and the behaviour of water at interfaces. Q Rev Biophys. 1985 Nov;18(4):323–422. doi: 10.1017/s0033583500005369. [DOI] [PubMed] [Google Scholar]
- Hamabata A., Von Hippel P. H. Model studies on the effects of neutral salts on the conformational stability of biological macromolecules. II. Effects of vicinal hydrophobic groups on the specificity of binding of ions to amide groups. Biochemistry. 1973 Mar 27;12(7):1264–1271. doi: 10.1021/bi00731a004. [DOI] [PubMed] [Google Scholar]
- Hermann R. B. Use of solvent cavity area and number of packed solvent molecules around a solute in regard to hydrocarbon solubilities and hydrophobic interactions. Proc Natl Acad Sci U S A. 1977 Oct;74(10):4144–4145. doi: 10.1073/pnas.74.10.4144. [DOI] [PMC free article] [PubMed] [Google Scholar]
- Lee B. The physical origin of the low solubility of nonpolar solutes in water. Biopolymers. 1985 May;24(5):813–823. doi: 10.1002/bip.360240507. [DOI] [PubMed] [Google Scholar]
- Lin T. Y., Timasheff S. N. On the role of surface tension in the stabilization of globular proteins. Protein Sci. 1996 Feb;5(2):372–381. doi: 10.1002/pro.5560050222. [DOI] [PMC free article] [PubMed] [Google Scholar]
- Livingstone J. R., Spolar R. S., Record M. T., Jr Contribution to the thermodynamics of protein folding from the reduction in water-accessible nonpolar surface area. Biochemistry. 1991 Apr 30;30(17):4237–4244. doi: 10.1021/bi00231a019. [DOI] [PubMed] [Google Scholar]
- Madan B., Lee B. Role of hydrogen bonds in hydrophobicity: the free energy of cavity formation in water models with and without the hydrogen bonds. Biophys Chem. 1994 Aug;51(2-3):279–289. doi: 10.1016/0301-4622(94)00049-2. [DOI] [PubMed] [Google Scholar]
- Makhatadze G. I., Privalov P. L. Protein interactions with urea and guanidinium chloride. A calorimetric study. J Mol Biol. 1992 Jul 20;226(2):491–505. doi: 10.1016/0022-2836(92)90963-k. [DOI] [PubMed] [Google Scholar]
- Melander W., Horváth C. Salt effect on hydrophobic interactions in precipitation and chromatography of proteins: an interpretation of the lyotropic series. Arch Biochem Biophys. 1977 Sep;183(1):200–215. doi: 10.1016/0003-9861(77)90434-9. [DOI] [PubMed] [Google Scholar]
- Miller S., Janin J., Lesk A. M., Chothia C. Interior and surface of monomeric proteins. J Mol Biol. 1987 Aug 5;196(3):641–656. doi: 10.1016/0022-2836(87)90038-6. [DOI] [PubMed] [Google Scholar]
- Nandi P. K., Robinson D. R. The effects of salts on the free energies of nonpolar groups in model peptides. J Am Chem Soc. 1972 Feb 23;94(4):1308–1315. doi: 10.1021/ja00759a043. [DOI] [PubMed] [Google Scholar]
- Nandi P. K., Robinson D. R. The effects of salts on the free energy of the peptide group. J Am Chem Soc. 1972 Feb 23;94(4):1299–1308. doi: 10.1021/ja00759a042. [DOI] [PubMed] [Google Scholar]
- Pace C. N., Vanderburg K. E. Determining globular protein stability: guanidine hydrochloride denaturation of myoglobin. Biochemistry. 1979 Jan 23;18(2):288–292. doi: 10.1021/bi00569a008. [DOI] [PubMed] [Google Scholar]
- Pratt L. R., Pohorille A. Theory of hydrophobicity: transient cavities in molecular liquids. Proc Natl Acad Sci U S A. 1992 Apr;89:2995–2999. doi: 10.1073/pnas.89.7.2995. [DOI] [PMC free article] [PubMed] [Google Scholar]
- Santoro M. M., Bolen D. W. Unfolding free energy changes determined by the linear extrapolation method. 1. Unfolding of phenylmethanesulfonyl alpha-chymotrypsin using different denaturants. Biochemistry. 1988 Oct 18;27(21):8063–8068. doi: 10.1021/bi00421a014. [DOI] [PubMed] [Google Scholar]
- Schellman J. A. A simple model for solvation in mixed solvents. Applications to the stabilization and destabilization of macromolecular structures. Biophys Chem. 1990 Aug 31;37(1-3):121–140. doi: 10.1016/0301-4622(90)88013-i. [DOI] [PubMed] [Google Scholar]
- Schellman J. A. Selective binding and solvent denaturation. Biopolymers. 1987 Apr;26(4):549–559. doi: 10.1002/bip.360260408. [DOI] [PubMed] [Google Scholar]
- Sharp K. A., Nicholls A., Fine R. F., Honig B. Reconciling the magnitude of the microscopic and macroscopic hydrophobic effects. Science. 1991 Apr 5;252(5002):106–109. doi: 10.1126/science.2011744. [DOI] [PubMed] [Google Scholar]
- Spolar R. S., Livingstone J. R., Record M. T., Jr Use of liquid hydrocarbon and amide transfer data to estimate contributions to thermodynamic functions of protein folding from the removal of nonpolar and polar surface from water. Biochemistry. 1992 Apr 28;31(16):3947–3955. doi: 10.1021/bi00131a009. [DOI] [PubMed] [Google Scholar]
- VONHIPPEL P. H., WONG K. Y. NEUTRAL SALTS: THE GENERALITY OF THEIR EFFECTS ON THE STABILITY OF MACROMOLECULAR CONFORMATIONS. Science. 1964 Aug 7;145(3632):577–580. doi: 10.1126/science.145.3632.577. [DOI] [PubMed] [Google Scholar]
- Von Hippel P. H., Peticolas V., Schack L., Karlson L. Model studies on the effects of neutral salts on the conformational stability of biological macromolecules. I. Ion binding to polyacrylamide and polystyrene columns. Biochemistry. 1973 Mar 27;12(7):1256–1264. doi: 10.1021/bi00731a003. [DOI] [PubMed] [Google Scholar]
- Wada A. The alpha-helix as an electric macro-dipole. Adv Biophys. 1976:1–63. [PubMed] [Google Scholar]