Abstract
Recombinant and purified Thermotoga maritima CopA sustains ATPase velocity of 1.78–2.73 μmol/mg/min in the presence of Cu+ (pH 6, 60 °C) and 0.03–0.08 μmol/mg/min in the absence of Cu+. High levels of enzyme phosphorylation are obtained by utilization of [γ-32P]ATP in the absence of Cu+. This phosphoenzyme decays at a much slower rate than observed with Cu·E1 ∼ P. In fact, the phosphoenzyme is reduced to much lower steady state levels upon addition of Cu+, due to rapid hydrolytic cleavage. Negligible ATPase turnover is sustained by CopA following deletion of its N-metal binding domain (ΔNMBD) or mutation of NMBD cysteines (CXXC). Nevertheless, high levels of phosphoenzyme are obtained by utilization of [γ-32P]ATP by the ΔNMBD and CXXC mutants, with no effect of Cu+ either on its formation or hydrolytic cleavage. Phosphoenzyme formation (E2P) can also be obtained by utilization of Pi, and this reaction is inhibited by Cu+ (E2 to E1 transition) even in the ΔNMBD mutant, evidently due to Cu+ binding at a (transport) site other than the NMBD. E2P undergoes hydrolytic cleavage faster in ΔNMBD and slower in CXXC mutant. We propose that Cu+ binding to the NMBD is required to produce an “active” conformation of CopA, whereby additional Cu+ bound to an alternate (transmembrane transport) site initiates faster cycles including formation of Cu·E1 ∼ P, followed by the E1 ∼ P to E2-P conformational transition and hydrolytic cleavage of phosphate. An H479Q mutation (analogous to one found in Wilson disease) renders CopA unable to utilize ATP, whereas phosphorylation by Pi is retained.
Cation transport ATPases utilize ATP-free energy for transport of specific ions across biological membranes, against electrochemical gradients. They are referred to as P-type ATPases when enzyme phosphorylation, obtained by phosphoryl transfer from ATP to the enzyme protein, is an obligatory intermediate step in the mechanism of ATP utilization and coupled cation transport (1–3). The P-type ATPase family is divided into five branches referred to as I–V (4), including the important PII-type ATPases, which are specific for H+, Na+, K+, and Ca2+, and a PIB subgroup that includes ATPases for transport of heavy metals ions such as Cu+, Cu2+, Zn2+, Pb2+, Cd2+, and Co2+ (5). The PIB ATPases sustain important roles in accumulation and tolerance of heavy metal in biological systems (6–8), as well as for delivery of copper to metalloenzymes (9). Two ATPases of this subgroup serve as copper transporters in humans (10). Mutations of these proteins are involved in the etiology of Menkes and Wilson diseases (8, 11–13). The catalytic mechanism of the PIB ATPases has been the subject of preliminary studies (14–19).
For a comparative evaluation, it is useful to consider that the structure of PII-type ATPases, originally established for the Ca2+-ATPase by sequence analysis (20) and crystallography (21), includes three cytosolic domains referred to as N (nucleotide binding), P (phosphorylation), and A (actuator) domains, and 10 transmembrane helices containing the specific cation binding site for catalytic activation and transport. On the other hand, the structure of the PIB Cu+-ATPases has been determined only for the cytosolic region (22–24). Nevertheless, analogy with the PII ATPases structure has been clearly demonstrated (25), including three cytosolic domains and 8 (rather than 10) transmembrane segments. The 4th to 8th transmembrane segments bear resemblance to the corresponding Ca2+ ATPase segments, but the 1st to 3rd are rather different. A specific feature of the PIB ATPases is the presence of an N-terminal segment containing GMXCXXC motifs (26) that are evidently able to bind heavy metals and may serve as acceptors for metallochaperone-bound copper (27–29) and/or may have a copper-dependent regulatory role on enzyme activity (30).
The copper ATPase of the thermophilic Archaeoglobus fulgidus has proved to be a convenient protein for mechanistic studies (31–33), due to its heterologous expression and purification, and its stability. Expression and purification of a CopA homolog from Thermotoga maritima (34) (NCB accession number AE000512) was previously reported, including proteolytic digestion studies of domain organization and movements concomitant with catalytic activation (35). The protein comprises 726 amino acids, and includes only a single CXXC motif at its N-terminal (NMBD).2 We have now performed detailed measurements of intermediate phosphorylation reactions with ATP or Pi as substrates, using WT CopA, protein with NMBD deletion, protein with CXXC mutation, and also protein with a H479Q mutation, which is analogous (36) to a mutation reported to occur in Wilson disease.
MATERIALS AND METHODS
Preparations of Proteins
Overexpression of T. maritima CopA (TM0317) in Escherichia coli and purification of the expressed protein was previously described (35). The CopA constructs used in this study are shown in Fig. 1. The purified protein was suspended in a buffer containing 0.5 m NaCl, 20% (w/v) glycerol, and 50 mm MOPS/NaOH, pH 7.0, and stored at -80 °C. For all experiments the protein (0.5 mg/ml) was reduced by exposure (1 h on ice) to 2 mm DTT in 20 mm MOPS, pH 7, 20% glycerol, and 0.01% DDM. Sarcoplasmic reticulum vesicles containing the SERCA1 isoform of the Ca2+-ATPase were obtained from rabbit skeletal muscle as described by Eletr and Inesi (37).
FIGURE 1.
CopA constructs used in our experiments. Full-length CopA (WT, CXXC mutant, H479Q mutant) consists of 762 residues including a 36-residue extension (MGGSHHHHHHGMASMTGGQQMGRDLYDDDDKDPSSR) at the N terminus. The CXXC mutant has two substitutions (C27A and C30A). In the ΔNMBD, 90 residues (1Met–90Glu) were removed and a 10-residue extension (MDHHHHHHLE) was attached to the N terminus. The molecular weights are 83,361 (WT), 83,297 (CXXC mutant), 83,352 (H479Q mutant), 70,922 (ΔNMBD).
Preparation of Lipids
20 μl of egg yolk phosphatidylcholine (100 mg/ml in chloroform) was dried under vacuum. 1 ml of 10 mm MOPS, pH 7, was added and sonicated until disappearance of visible lipid particles.
ATPase Measurements
Reaction Mixture—The reaction mixture was 50 mm MES/triethanolamine, pH 6.0, 30% glycerol, 2.0 mm sodium azide, 0.5 mm DTT, 10 mm cysteine/Tris, pH 6.0, 5 mm MgCl2, 0.01% DDM, 25 μg/ml phosphatidylcholine and either 1 mm BCS or added CuCl2 (40 μm in most experiments).
Procedure—We added 25 μl of reduced protein to 450 μl of reaction mixture (with 1 mm BCS or 40 μm CuCl2). The final concentration was 25 μg/ml with a temperature of 60 °C. The reaction was started by addition of 25 μl of 50 mm ATP (2.5 mm final concentration). 50-μl samples at 30, 60, 90, 120, and 150 s for colorimetric Pi determination (38) were removed.
Phosphorylation with ATP
Reaction Mixture—The reaction mixture was 50 mm MES/triethanolamine, pH 6.0, 30% glycerol, 0.5 mm DTT, 10 mm cysteine/Tris, pH 6.0, 5 mm MgCl2, 0.01% DDM, 25 μg/ml phosphatidylcholine and either 1 mm BCS or 40 μm CuCl2.
Procedure—To 900 μl of reaction mixture (with 1 mm BCS or 40 μm CuCl2) at 60 °C was added 50 μl of 0.5 mm [γ-32P]ATP (25 μm final concentration). To the mixture we added 50 μl of reduced protein and quenched the solution with 5 ml of 3% trichloroacetic acid and then filtered the mixture. The incubation times were 0, 5, 10, 20, 30, 60, 120, and 300 s.
Alternatively, 50 μl of reduced protein was added to 900 μl of reaction mixture (with 1 mm BCS or 40 μm CuCl2) at 60 °C. In some cases, this mixture was incubated with 1 mm BCS for 3 h at 37 °C. Subsequently, the mixture was transferred to a bath at 60 °C, and 50 μl of 0.5 mm [γ-32P]ATP was added (25 μm final concentration) and quenched with 5 ml of 3% tricholoroacetic acid and then filtered. The incubation times were 0, 5, 10, 20, 30, 60, 120, and 300 s.
The quenched samples were passed through 0.45-μm Millipore filters, which were then blotted and processed for radioactivity determination by liquid scintillation counting. For electrophoretic analysis of the phosphorylated protein, the quenched samples were centrifuged at 5,000 × g for 20 min, and the sediments were dissolved in 2.5% lithium dodecylsulfate, 0.5% β-mercaptoethanol, 3% sucrose, and 0.1 mg of bromphenol blue/ml. An aliquot of the dissolved protein was subjected to gel electrophoresis at pH 6.3 (39), and the gels were finally stained with Coomassie Blue R-250, or scanned for phosphorimaging.
In some experiments the filtrate was treated with activated charcoal for removal of nucleotide, the charcoal was removed by filtration, and the [γ-32P]Pi in the supernatant was isolated by ammonium molybdate isobutyl alcohol/benzene extraction (40). An aliquot was then used for determination of [γ-32P]Pi by scintillation counting and assessment of ATPase hydrolytic activity in parallel with phosphoenzyme formation.
Phosphorylation with Pi
The reaction mixture was 50 mm MES/triethanolamine, pH 6.0, 20% Me2SO, 0.5 mm DTT, 10 mm cysteine/Tris, pH 6.0, 5 mm MgCl2, 0.01% DDM, 25 μg/ml phosphatidylcholine, and either 1 mm BCS or 40 μm CuCl2. Following incubation with 50 μm [32P]Pi for various times, the samples were quenched, filtered, and the phosphorylated protein on the filters was examined as described above.
Phosphoenzyme Decay
Maximal levels of phosphorylation were first obtained by preincubation with [γ-32P]ATP (25 μm final concentration) or [32P]Pi (50 μm final concentration) in the absence of Cu2+ or BCS. A chase with 1 mm nonradioactive ATP or Pi was then performed, and samples were quenched with acid at various time intervals and processed as described above for determination of residual [32P]phosphoenzyme.
Determination of Residual Bound Copper
To establish the efficiency of copper extraction from CopA with BCS, we incubated CopA protein with 1 mm BCS for 3 h at 37 °C, under the same conditions used for the phosphorylation experiments with ATP. We then removed the BCS by dialysis for 16 h with an intermediate dialysis medium change, and submitted the remaining protein for measurement of residual copper by ICP-MS.
Alternatively, CopA protein was incubated with 1 mm BCS as described above and 0.9 ml of this protein solution was supplemented with 200 μl of 20 mg/ml PC, centrifuged at 217,000 × g for 30 min, the supernatant discarded, and the pellet re-suspended in 1 ml of wash buffer (50 mm MES/triethanolamine, pH 6, 30% (w/v) glycerol, 10 mm cysteine, 5 mm MgCl2, and 0.5 mm DTT, 1 mm BCS). The resuspended sample was then centrifuged again at 217,000 × g for 30 min, the supernatant was discarded, and the pellet re-suspended in 100 μl of 100 mm MOPS/triethanolamine, pH 7. This protein mixture was submitted for measurement of residual copper by ICP-MS.
RESULTS
Steady State ATPase Activity—Determination of ATPase hydrolytic activity by colorimetric measurement of Pi production revealed that at 60 °C and pH 6.0, the WT enzyme sustained a steady state velocity of 1.78–2.73 μmol/mg of protein/min under conditions of maximal activation by Cu+ (assuming that Cu(I) is produced by the high concentrations of reducing agents in the reaction mixture) and 0.03–0.08 μmol/mg of protein/min in the absence of copper (Table 1). A graphic representation of ATPase activity in the absence of added copper (1 mm BCS present), or following addition of increasing concentrations of CuCl2 is given in Fig. 2. As shown in the figure, maximal activity is obtained in the presence of 5–40 μm (added) CuCl2, as previously reported (35). Considering the stoichiometry of CopA in our preparation (based on an Mr 83,352 for our CopA protein in a nearly pure preparation), the maximal steady state velocity corresponds to turnover numbers of ∼3.0-4.0 and 0.05–0.10 s-1, in the presence or absence of Cu+, respectively. The activity in the presence of Cu+ was ∼10-fold lower if the measurements were performed at pH 7 rather than 6, or at a temperature of 40 °C rather than 60 °C (Table 1).
TABLE 1.
Steady state ATPase activity of WT CopA at pH 6 or 7, in the presence of 40 μm CuCl2 or in the absence of Cu+ (1 mm BCS present)
pH | Temperature | Cu2+ | ATPase |
---|---|---|---|
°C | μm | μmol/mg/min | |
6 | 60 | 40 | 1.78–2.73 |
6 | 60 | 0.03–0.08 | |
6 | 40 | 40 | 0.23–0.26 |
6 | 40 | 0.004–0.005 | |
7 | 60 | 40 | 0.26–0.31 |
7 | 60 | 0.10–0.14 |
FIGURE 2.
Copper dependence of CopA steady state activity. ATPase activity was measured by colorimetric determination of Pi, at pH 6.0 and 60 °C (see “Materials and Methods”). As indicated in the figure, the measurements were obtained either in the presence of 1 mm BCS or in the absence of BCS following addition of incremental concentrations of CuCl2.
We then studied the ATP concentration dependence of CopA, in the presence of an ATP regenerating system (to maintain steady state activity in the presence of μm ATP) and at 40 °C (to prevent denaturation of the regenerating system at higher temperature). We found significant ATPase activity with ATP in the micromolar concentration range (see below phosphoenzyme formation), but much higher activity when the ATP concentration was raised to the millimolar level. This phenomenon was previously observed with SERCA (41) and attributed to turnover activation by low affinity ATP binding (42). We considered the possibility that, under steady state conditions and in the presence of micromolar concentrations, ATP regeneration may be only partially efficient. However, the same results were obtained even when the concentration of the ATP regenerating system was quadrupled. Double-reciprocal plots of the values obtained with SERCA at 37 °C yield Vmax = 6.25 μmol/mg/min and Km = 5.04 μm for the low range, and Vmax = 15.06 μmol/mg/min and Km = 48.24 μm for the high range (Fig. 3A). On the other hand, double-reciprocal plots of the values obtained with CopA at 40 °C yield Vmax = 32.4 nmol/min/mg and Km = 10.0 μm for the low range, and a Vmax = 425 nmol/min/mg and Km = 1.54 mm for the high range (Fig. 3B).
FIGURE 3.
Double-reciprocal plots of SERCA (A) and CopA (B) steady state hydrolytic activity as a function of ATP concentration. A, the reaction was started at 37 °C by addition of various concentrations of ATP to a medium containing 50 mm KCl, 50 mm MOPS, pH 7, 80 mm KCl, 3 mm MgCl2, 20 μm CaCl2, 1 μm A12387 (Ca2+ ionophore), 1 mm phosphoenolpyruvate, 50 g/ml pyruvate kinase, and 30 μg of SR protein/ml. B, the reaction was started at 40 °C by addition of various concentrations of ATP to a medium containing 50 mm MES/triethanolamine, pH 6.0, 30% glycerol, 2.0 mm sodium azide, 0.5 mm DTT, 10 mm cysteine/Tris, pH 6.0, 5 mm MgCl2, 0.01% DDM, 25 μg/ml phosphatidylcholine, 40 μm CuCl2, 1 mm phosphoenolpyruvate, 50 g/ml pyruvate kinase, and 25 μg of reduced CopA protein/ml. Samples were taken at various time intervals for colorimetric determination of Pi, whereby linear plots of steady state velocity were obtained. The unit of velocity is nanomole/mg/min.
The steady state ATPase turnover (at 60 °C) of the ΔNMBD protein (i.e. deprived of N terminus Cu+ binding sequence) was very low in the presence (0.24 μmol/mg/min) or absence of copper. Furthermore, the turnovers of CXXC (mutations of NMBD cysteines involved in copper binding) and H479Q (corresponding to a mutation of the copper ATPase ATP7B in Wilson disease (36)) were even lower (data not shown).
Phosphorylation by Utilization of ATP—As previously observed with the A. fulgidus CopA (31, 33), we find that the T. maritima CopA is phosphorylated by ATP. Depending on the preparation of WT protein, the maximal levels of phosphoenzyme obtained in our experiments vary between 5 and 10 nmol/mg of protein. These levels are much higher than obtained previously and (considering an 83 kDa for the purified CopA preparation) nearly match the stoichiometry of the ATPase protein. We find that when [γ-32P]ATP (25 μm final concentration) is added to WT protein in the absence of Cu+ (1 mm BCS present), acid stable phosphorylation reaches maximal levels with an approximate half-time of5sat60 °C (Fig. 4A). For the same preparation, the maximal phosphoenzyme levels were 10–15% higher if the protein was preincubated for 3 h with 1 mm BCS at 37 °C, before transfer to a 60 °C bath and addition of [γ-32P]ATP. To exclude the presence of residual copper in significant quantity, we incubated CopA protein with 1 mm BCS for 3 h at 37 °C, under the same conditions used for the phosphorylation experiments with ATP. Then we removed the BCS as described under “Materials and Methods,” and submitted the remaining protein for measurement of residual copper by ICP-MS. A water sample run as a control yielded no detectable level. A copper standard run at the same time yielded 97% of the expected value. The copper levels found in different preparations of CopA protein treated with BCS were hardly above the minimal detectable signal, and varied between 0.0001 and 0.02 mol of copper per mol of CopA. Considering the unavoidable variability of hardly detectable levels, we conclude that the residual copper in samples treated with BCS is negligible. This is in agreement with functional experiments, demonstrating formation of E2-P by utilization of Pi, which is then inhibited by addition of copper (see below, Fig. 9).
FIGURE 4.
Phosphoenzyme formation by utilization of ATP. A, the reaction was started by addition of 25 μm [γ-32P]ATP to WT CopA in the presence of 1 mm BCS (absence of Cu+) (•), with 40 μm CuCl2 added in conjunction with [γ-32P]ATP (○), or by addition of [γ-32P]ATP following a 2-min preincubation with 40 μm Cu2+ (▾). Temperature was 60 °C. B, initial velocity of phosphoenzyme formation as a function of ATP concentration. CopA was preincubated for 2 min with 1 mm BCS before addition of [γ-32P]ATP. Temperature was 40 °C. See “Materials and Methods” for reaction mixtures.
FIGURE 9.
Phosphorylation of WT CopA with Pi and subsequent decay. A, phosphorylation was started by the addition of 50 μm [32P]Pi to WT CopA in conjunction with 1 mm BCS (•) or 40 μm CuCl2 (○), or following a 2-min preincubation with 40 μm CuCl2 (▾). B, Pi concentration dependence of the phosphoenzyme equilibrium level, in the presence of 1 mm BCS. Incubation was for 5 min at 60 °C. C, decay was started with a 1 mm non-radioactive Pi chase added to [32P]phosphoenzyme obtained by a 5-min incubation with 50 μm [32P]Pi in the absence of both BCS and CuCl2. The chase medium contained either 1 mm BCS (•) or 40 μm CuCl2 (○). The temperature was 60 °C.
If [γ-32P]ATP was added to WT protein preincubated with Cu+, a phosphorylation level of 1 nmol/mg of protein was reached within 2 s (Fig. 4A). However, such a low level did not increase any further, suggesting that a low steady state level of phosphoenzyme was determined by Cu+ activation of hydrolytic cleavage. In fact, if [γ-32P]ATP and copper were added simultaneously to the WT protein, a relatively high level of enzyme phosphorylation was obtained within 30 s, but the phosphoenzyme level decayed thereafter (Fig. 4A). This reduction is evidently due to activation of the hydrolytic cleavage by Cu+, whereby lower steady state levels of phosphoenzyme are established.
The ATP concentration dependence of phosphoenzyme formation was determined by measuring initial velocities at 40 °C and in the absence of Cu+. A plot of the phosphorylation velocity versus ATP concentration (Fig. 4B) yields a Vmax value of 0.465 nmol/s (∼30 nmol/min) per mg of protein (at 40 °C and in the absence of Cu+), with an apparent Km value of 9.05 μm. A similar Km value was obtained by Mandal et al. (31) for phosphorylation of A. fulgidus CopA under conditions of silver activation. In agreement with these findings, Rice et al. (33) obtained maximal levels of phosphorylation using ATP concentrations within the micromolar range. It should be pointed out that the phosphoenzyme levels obtained in our experiments with T. maritima CopA (in the absence of Cu+) are 4–5-fold higher than those observed by us in the presence of Cu+, and reported for A. fulgidus CopA using silver activation (31). In addition, we found that, whereas phosphoenzyme formation and ATPase activity in the presence of copper were highly dependent on the presence of millimolar Mg2+, the rate of phosphoenzyme formation in the absence of copper was faster in the presence of Mg2+, but high levels of phosphoenzyme could be obtained even in the absence of Mg2+, albeit at a slower rate (data not shown).
To ensure that the radioactive protein measured in the phosphorylation experiments corresponded only to the ATPase, we examined the phosphoenzyme on electrophoretic gels, comparing the protein stain and autoradiography of WT SERCA and WT CopA following reaction with [γ-32P]ATP (Fig. 5). Reassuringly, the radioactive band is identified with the enzyme protein band in both cases. Furthermore, it is clear that Ca2+ is an absolute requirement for SERCA phosphorylation, whereas Cu+ is not required for phosphorylation of CopA. In fact, the CopA radioactive band is much weaker in the presence than in the absence of Cu+.
FIGURE 5.
Electrophoretic analysis of Ca2+-ATPase (SERCA) and CopA following phosphorylation with [γ-32P]ATP. The gels were either stained with Coomassie Blue to evidence protein bands, or scanned with a phosphorimager to detect radioactive phosphoenzyme. The reaction with 25 μm [γ-32P]ATP was allowed to proceed under conditions yielding the highest steady state levels of phosphorylation for each protein. SERCA, 5 s in ice in the presence of 50 mm MOPS, pH 7, 80 mm KCl, 1 mm MgCl2, and 20 μm CaCl2 or 1 mm EGTA. CopA, 3 min at 60 °C, in the presence of 50 mm MES/triethanolamine, pH 6.0, 30% glycerol, 0.5 mm DTT, 10 mm cysteine/Tris, pH 6.0, 5 mm MgCl2, 0.01% DDM, 25 μg/ml phosphatidylcholine, and either 1 mm BCS or 40 μm CuCl2. See “Materials and Methods” for details.
Because the experiments illustrated in Figs. 4 and 5 suggest that the steady state level of the WT CopA phosphoenzyme obtained in the absence of copper is reduced by addition of copper through activation of its hydrolytic cleavage, we compared the pre-steady state behavior of SERCA and CopA upon sequential addition of ATP and activating cation. It is shown in Fig. 6A that addition of [γ-32P]ATP to SERCA in the absence of Ca2+ yields neither phosphoenzyme nor Pi. A sudden burst of phosphoenzyme is then obtained upon addition of Ca2+, followed by steady state Pi production. On the contrary, addition of [γ-32P]ATP to CopA in the absence of Cu+ (Fig. 6B) is followed immediately by phosphoenzyme formation, but no Pi production. Addition of Cu+ then yields a Pi burst concomitant with a conspicuous reduction of the phosphoenzyme, followed by steady state Pi production and a lower EP level.
FIGURE 6.
Pre-steady state behavior of SERCA (A) and CopA (B) upon sequential additions of ATP and Ca2+ or Cu+·SERCA (0.05 mg/ml) preincubated for 15 min in a medium containing 50 mm MOPS, pH 7, 80 mm KCl, 1 mm MgCl2, 1 μm A23187 (Ca2+ ionophore), and 1 mm EGTA. The reaction was started at 10 °C by the addition of 25 μm [γ-32P]ATP and, after 10 s, 1.05 mm CaCl2 was added. CopA (0.1 mg/ml) was preincubated at 37 °C with 0.1 mm BCS for 2 h, in a reaction mixture as described under “Materials and Methods.” The reaction was started at 60 °C by the addition of 25 μm [γ-32P]ATP and, after 10 s, 0.14 mm CuCl2 was added. Samples were taken for determination of phosphoenzyme (•) and Pi (○) at the time intervals as indicated. Each time point shown in the graph is the average of three different determinations. Note the Pi burst in concomitance with EP reduction upon addition of copper.
With regard to the behavior of mutants, we found that when [γ-32P]ATP is added to the ΔNMBD protein, phosphorylation occurs at a very fast rate (rate constant > 1 s-1) either in the absence or presence of Cu+, reaching levels (8–12 nmol/mg of protein, depending on the preparation) that nearly match the stoichiometry of the enzyme in the reaction mixture (Fig. 7A). Similarly, the CXXC mutant is phosphorylated by [γ-32P]ATP in the absence or presence of Cu+ (Fig. 7B), but at a significantly lower velocity. These high levels of phosphorylation of either ΔNMBD protein or CXXC with ATP are not at all affected by Cu+. It is apparent that phosphorylation is considerably faster in the absence (i.e. ΔNMBD) than in the presence of the NMBD segment that is unable to bind Cu+. The levels of phosphorylation (less than 1 nmol/mg of protein) that are obtained with the H479Q mutant are very low (not shown), suggesting that this mutation interferes with ATP binding and/or utilization (36).
FIGURE 7.
Phosphorylation of ΔNMBD (A) and CXXC(B) by utilization of ATP. The reaction was started by addition of 25 μm [γ-32P]ATP to ΔNMBD (A) or CXXC(B). The protein was preincubated with either 1 mm BCS (○) or 40 μm CuCl2 (•). See “Materials and Methods” for reaction mixtures. The temperature was 60 °C.
If the phosphoenzyme obtained with WT protein by utilization of [γ-32P]ATP in the absence of copper or BCS is chased with an excess (1 mm) of nonradioactive ATP, the radioactive phosphoenzyme decays with a 0.05 s-1 rate constant at 60 °C (Fig. 8A). However, if the chase is done with ATP and Cu+ (40 μm CuCl2 added), the phosphoenzyme level decays in a shorter time (0.3 s-1 rate constant: Fig. 8A), indicating activation of hydrolytic cleavage by Cu+. However, this rate constant is lower (see “Discussion”) than the 3 s-1 turnover observed under steady state conditions in the presence of Cu+ (Table 1).
FIGURE 8.
Decay of phosphoenzyme obtained with ATP. The phosphoenzyme was obtained by a 3-min incubation with 25 μm [γ-32P]ATP (temperature, 60 °C), using WT (A), ΔNMBD (B), or CXXC (C) CopA, without preincubation with Cu+ or BCS. A chase was then started by addition of 1 mm non-radioactive ATP, in conjunction with 1 mm BCS (○) or 40 μm CuCl2 (•). The decay reaction was acid quenched at various times as indicated.
Decay of the phosphoenzyme obtained with the ΔNMBD protein or the CXXC mutant by utilization of [γ-32P]ATP in the absence of Cu+ or BCS is not accelerated by Cu+ (Fig. 8, B and C), and proceeds at a lower rate in the case of the CXXC mutant. This demonstrates that Cu+ binding to the NMBD is required to activate decay of the phosphoenzyme obtained with ATP (compare Fig. 8, A with B and C). In addition, it is apparent that the presence of the mutated NMBD (i.e. CXXC mutant) slows down phosphoenzyme cleavage.
Phosphorylation by Utilization of Pi—Phosphorylation of the Ca2+ transport ATPase can be obtained by reversal of the hydrolytic reaction that normally ends the catalytic cycle (43). This reaction occurs only in the absence of Ca2+ (i.e. ATPase in the E2 state), and is inhibited by Ca2+ (i.e. the E1 state). Similarly, we found that if [32P]Pi is added to WT CopA in the absence of Cu+ (BCS present), enzyme phosphorylation reaches maximal levels of 4.5–5.0 nmol/mg of protein with an approximate 0.03 s-1 rate constant (Fig. 9A). A plot of the phosphoenzyme levels obtained following equilibration in the presence of increasing concentrations of Pi, and in the absence of Cu+, yields EPmax = 5.6 nmol/mg of protein and Kd = 3.3 × 10-6 m (Fig. 9B). These values were obtained in the presence of Me2SO, and are likely to be less favorable under physiological conditions. It is noteworthy that the maximal levels of phosphoenzyme obtained with Pi are generally lower than obtained with ATP. This is due to the equilibrium character of the Pi experiments, with a constant yielding phosphorylation of approximately half of the protein available. On the other hand, the steady state character of the ATP reaction pushes the phosphoenzyme to the limit permitted by the rates of phosphorylation and hydrolytic cleavage. If [32P]Pi is added in conjunction with CuCl2 or the enzyme is preincubated for 2 min with CuCl2, the phosphorylation level is significantly lower (Fig. 9A), suggesting a Cu+-dependent activation of phosphoenzyme cleavage and/or a conformational change (E2 to E1) that interferes with enzyme phosphorylation by Pi.
Chase of the [32P]phosphoenzyme with non-radioactive Pi (Fig. 9C) in the absence of Cu+ yields a slow decay of the phosphoenzyme level with a 0.015 s-1 rate constant. Addition of CuCl2 with the non-radioactive Pi, however, yields much faster phosphoenzyme decay, evidently due to a Cu+-induced conformational change that affects the rate of phosphoenzyme cleavage. This effect of copper is evidently due to binding to the NMBD, because no stimulation by copper is observed with E2-P obtained with ΔNMBD protein (see below Fig 10B; see also “Discussion” and Scheme 1).
FIGURE 10.
Phosphorylation of ΔNMBD protein with Pi and subsequent decay. A, phosphorylation was started by the addition of 50 μm [32P]Pi to ΔNMBD in conjunction with either 1 mm BCS (•) or 40 μm CuCl2 (○), or following a 2-min preincubation with 40 μm CuCl2 (▾). B, decay was started with a 1 mm non-radioactive Pi chase added to [32P]phosphoenzyme obtained by a 5-min equilibration with 50 μm [32P]Pi in the absence of both BCS and CuCl2. The chase medium contained either 1 mm BCS (•) or 40 μm Cu2+ (○). The temperature was 60 °C.
SCHEME 1.
The diagram suggests that, in the absence of Cu+, CopA resides in a basal (Eb) conformation that utilizes ATP or Pi at slow rates. Cu+ binding to the NMBD induces transition to an active CopA conformation (Ea), whereby additional Cu+ binding to an alternate, transmembrane metal binding (TM-MBS) transport site allows faster cycles, including the E1 to E2 conformational transition, faster phosphoenzyme formation and cleavage, and most importantly transition of Ea1 ∼ P·Cu+ to Ea2 ∼ P·Cu+. Whereas binding and dissociation of the latter Cu+ occurs within each fast cycle, CopA activation by Cu+ binding to NMBD occurs with a time constant apparently slower than a single cycle of the activated enzyme.
Phosphorylation of the ΔNMBD protein by [32P]Pi occurs very rapidly (Fig. 10A), in analogy to its phosphorylation by [32P]ATP (Fig. 7A). However, whereas phosphorylation of the ΔNMBD protein by [32P]ATP is not influenced by Cu+ (Fig. 7A), phosphorylation by [32P]Pi is significantly affected, showing a slow reduction if CuCl2 is added in conjunction with [32P]Pi, and a very low initial level of phosphorylation if the protein is preincubated for 2 min with CuCl2 before addition of [32P]Pi (Fig. 10A). This is a very important finding, indicating that Cu+ induces a conformational change (E2 to E1) that interferes with enzyme phosphorylation by Pi, even though the ΔNMBD copper binding site is deleted, and even though the catalytic activation by Cu+ does not occur in the ΔNMBD protein. Therefore, an alternate transmembrane site (26) is operative in the absence of NMBD, and its occupancy by Cu+ induces the E2 to E1 transition. It is noteworthy that Gonzales-Guerrero and Arguello (44) recently reported copper binding measurements, indicating that the CopZ chaperone can transfer Cu+ to A. fulgidus CopA deprived of the NMBD. Our experiments agree with this report, and demonstrate that such binding does in fact involve the actual transport site (TMBS), inducing the E2 to E1 transition. It should be pointed out that the CopA protein is used in the presence of detergent and, therefore, such a copper site is likely to be exposed to the medium even when the enzyme resides in the E2 conformation. Apparently, under these conditions, Cu+ can reach the transport site even in the absence of CopZ, albeit at a slower rate.
In related chase experiments (Fig. 10B), hydrolytic cleavage of the phosphoenzyme obtained with Pi and the ΔNMBD protein is quite fast, and proceeds directly from E2-P to E2 + Pi with no effect of copper. Note that in this experiment we measure only decay of already formed [32P]E2-P, whereas no [32P]E2-P is formed that may be affected by re-equilibration of E2 to E1 following the addition of Cu2+ in conjunction with the nonradioactive Pi chase.
Phosphorylation of the CXXC mutant by Pi occurs at a slow rate (Fig. 11A), and is affected very slowly by copper even if the protein is preincubated for 2 min with copper before addition of [32P]Pi. Cleavage of the phosphoenzyme also occurs very slowly, and is not affected by copper (Fig. 11B). It is therefore apparent that the presence of the NMBD segment produces kinetic inhibition (compare with Fig. 10), which is only relieved if copper is allowed to bind.
FIGURE 11.
Phosphorylation of CXXC protein with Pi, and subsequent decay. A, phosphorylation was started by the addition of 50 μm [32P]Pi to the CXXC mutant in the presence of either 1 mm BCS (•) or 40 μm CuCl2 (○), or following a 2-min preincubation with 40 μm Cu2+ (▾). B, decay was started by addition of 1 mm non-radioactive Pi chase to [32P]phosphoenzyme obtained by a 5-min equilibration with 50 μm [32P]Pi in the absence of both BCS and CuCl2. The chase medium contained either 1 mm BCS (•) or 40 μm Cu2+ (○). The temperature was 60 °C.
Finally, we found that phosphorylation of the H479Q mutant with [32P]Pi proceeds in the absence or presence of copper (Fig. 12A) essentially as that of the WT in the absence of copper (Fig. 9A). This demonstrates that the lack of H479Q mutant phosphorylation by ATP is due to interference of the H479Q mutation with ATP positioning within the phosphorylation site (36), rather than the catalytic chemistry of phosphorylation. In fact, phosphorylation by Pi, its cleavage (Fig. 12B), and sensitivity to copper are normal in the H479Q mutant.
FIGURE 12.
Phosphorylation of H479Q mutant with Pi, and subsequent decay. A, phosphorylation was started by the addition of 50 μm [32P]Pi to H479Q in conjunction with either 1 mm BCS (•) or 40 μm CuCl2 (○). B, decay was started with a 1 mm non-radioactive Pi chase added to [32P]phosphoenzyme obtained by a 5-min equilibration with 50 μm [32P]Pi in the absence of both BCS and CuCl2. The chase medium contained either 1 mm BCS (•) or 40 μm CuCl2 (○). The temperature was 60 °C.
DISCUSSION
Preparation of T. maritima CopA by heterologous expression and subsequent purification (35) provides a very active enzyme that sustains maximal steady state ATPase activity at 60 °C and pH 6 (Fig. 2 and Table 1), with nearly total dependence on activation by copper and a turnover of 3–4 s-1. Unexpectedly, we found that acid-stable CopA phosphoenzyme can be formed by utilization of ATP in the absence as well as in the presence of copper. In fact, the steady state level of phosphoenzyme is much higher in the absence than in the presence of copper, due to ATPase activation by Cu+ and consequent phosphoenzyme cleavage.
The behavior of CopA is quite different from that of the Ca2+ ATPase (SERCA), whose phosphorylation by ATP is absolutely dependent on Ca2+ (Fig. 6A). It should be pointed out that the maximal velocity of CopA phosphorylation in the absence of Cu+ (30 nmol/min per mg of protein at 40 °C) is too slow to account for the steady state ATPase velocity observed in the presence of Cu2+ (∼350 nmol/mg/min of protein at 40 °C). It is therefore apparent that our preparation of CopA can be phosphorylated by ATP either in a “basal” (Eb) or in an “active” (Ea) conformational state, but only the active (Ea) conformation allows the fast turnover observed in the presence of Cu+ (Scheme 1). In fact, [32P]phosphoenzyme decay following a chase with nonradioactive ATP in the absence of copper (Fig. 8A) occurs with a rate constant of 0.05 s-1, matching the 0.05–0.10 s-1 turnover s-1 observed under steady state conditions in the absence of copper (Table 1). Furthermore, phosphoenzyme decay following addition of non-radioactive ATP in conjunction with copper (Fig. 8A) occurs with a 0.3 s-1 rate constant, which is still significantly lower than the 3–4 s-1 turnover observed under steady state conditions in the presence of copper (Table 1). This rather slow turnover is likely to be related to a time-dependent conformational effect of Cu+ binding to the NMBD, and the related conversion of the enzyme from basal to active conformation (Scheme 1). Finally, this conversion is also apparent if we consider that the high levels of phosphoenzyme obtained by utilization of ATP in the absence of copper are reduced to a much lower steady state upon addition of copper, due to hydrolytic cleavage as demonstrated by a surge of Pi production (Fig. 6B).
The ΔNMBD protein and the CXXC mutant can be phosphorylated by ATP equally well in the absence or presence of copper, and the phosphoenzyme level so obtained is not reduced by addition of copper to these mutants. Therefore the ATPase active state is obtained through Cu+ binding to the NMBD (Scheme 1). It is noteworthy that, in addition to the lack of copper effect, the rates of phosphorylation and cleavage are faster (Figs. 4, 7, and 8) in the absence (ΔNMBD protein) than in the presence of the NMBD sequence (WT protein or CXXC mutant). This suggests that the NMBD segment is an integral part of the enzyme headpiece (A domain) conformation, resulting in kinetic inhibition of the ATPase cycle when copper is absent. For this reason, Cu+ binding to the NMBD plays an essential role in inducing the activated state of WT CopA.
In analogy to SERCA (43), WT CopA can be phosphorylated with Pi (Fig. 9A). The resulting phosphorylation level is reduced by copper, likely due to hydrolytic cleavage as well as conversion of E2 to a Cu-E1 conformation that is not reactive to Pi. The E2-P formed with Pi undergoes slow cleavage (Fig. 9C) in the absence of copper, but its cleavage rate constant is increased by the addition of copper, again suggesting a Cu+-induced transition from Eb-P to Cu·Ea2-P (Scheme 1).
It is shown in Fig. 10A that the ΔNMBD protein can also be phosphorylated with Pi. Here again copper produces a slow transition whereby the phosphorylation level is reduced. This is a most interesting finding, demonstrating that in the absence of the NMBD, Cu+ is still effective and evidently binds to an alternate transmembrane (transport) site whose occupancy by Cu+ affects the Pi reaction even in the ΔNMBD protein. Because the ΔNMBD mutant sustains hydrolytic cleavage of E2-P (Fig. 10B), but does not sustain hydrolytic cleavage of ATP, it is apparent that the NMBD segment and its activation by copper are involved in E1 ∼ P formation by utilization of ATP, and are absolutely required for the E1 ∼ Pto E2-P transition in the ATPase cycle of the active enzyme (Ea in Scheme 1). The NMBD connection to the A domain suggests that NMBD has a strong influence on A domain movements and the proper positioning of its catalytically active residues in proximity to the phosphorylation site (P domain).
If we align the amino acid sequences involved in the phosphorylation reaction, we find homologies through the haloacid halogenase superfamily (45), including the phosphoserine phosphatase, T. maritima CopA, A. fulgidus CopA, sarcoplasmic Ca2+-ATPase, and renal Na+/K+-ATPase (Fig. 13). However, the consensus sequence for the TGES signature (A domain) is specifically limited to the transport ATPases, and does not include the phosphoserine phosphatase, as also demonstrated by structural studies (46, 47). This homology is related to the specific and prominent role sustained by the A (“actuator”) domain in the catalytic and transport mechanism of P ATPases, as the A domain lends itself to rotation and bending. These movements allow sequential interactions with the P and N domains, displacement of transmembrane segments that affect the cation transport sites, and proper placement of the TGES signature for catalytic processing of the phosphorylated intermediate (35, 48). It is of interest that, with the exception of the TGE motif, the structure of Cu-ATPases is not identical to the A domain of other P-type pumps (2, 10), due to the absence of an external loop region (24), and a lack of correspondence with the first half of the SERCA A domain (25). In addition, CopA presents eight (rather than 10) transmembrane segments, of which the 4th to 8th bear similarity to M2 to M6 of the Ca2+-ATPase and the Na+/K+-ATPase, whereas the 1st to 3rd are rather specific (25, 35). Finally, and most importantly, the A domain-linked NMBD, whose occupancy by copper is an absolute requirement for catalytic turnover, is a unique feature of CopA. These differences suggest that, in the absence of copper, CopA resides in a conformational state (not observed in the PII ATPases), whereby proper interaction of headpiece domains and catalytic turnover is not permitted. This state (“basal state” in Scheme 1) allows a slow rate of ATP utilization to form a phosphoenzyme with a conformation that is unable to proceed through the catalytic and transport cycle. Transition to the active state is then induced by copper binding to the NMBD through a relatively slow process (see reduction of phosphoenzyme level upon simultaneous addition of ATP and CuCl2 in Fig. 4A). Cu+ binding to the transmembrane metal binding domain (TM-MBD in Scheme 1) of the active enzyme then allows fast cycles, including the Cu+·E1 ∼ Pto E2-P conformational transition. We demonstrate here that Cu+ binding to the TM-MBD can occur even in the absence of Cu+ in the NMBD, with some conformational effect on the phosphorylation site, but without triggering the catalytic cycle. That is because copper binding to the NMBD is required to obtain the active conformation that sustains catalytic and transport cycles. This is a unique requirement of the copper ATPase, with no equivalent in the PII ATPases. The related conformational adjustment seems to involve mostly the A domain.
FIGURE 13.
Alignment of sequences involved in the phosphorylation reaction in the haloacid dehalogenase family. From the top, these sequences correspond to Pho-Ser-Pho, phosphoserine phosphatase from Methanococcus jannaschii; CopA from T. maritima; CopA from A. fulgidus; rabbit skeletal muscle sarcoplasmic Ca2+-ATPase; and porcine renal Na+/K+-ATPase.
An additional kinetic effect (not considered by the diagram in Scheme 1) is produced by low affinity ATP binding, as a higher steady state velocity is observed in the presence of millimolar ATP, whereas the phosphorylated intermediate is formed by micromolar ATP (Fig. 3B). This phenomenon was also observed with SERCA (41), and attributed to low affinity ATP binding, whereby the rate of E2 to E1 transition is increased (42), including dissociation of exchange H+ from cation binding sites (49).
Finally, our experiments demonstrate that the H479Q mutation of CopA produces total inhibition of phosphoenzyme formation with ATP and steady state ATPase activity. On the other hand, phosphorylation with Pi and its hydrolytic cleavage proceed normally, indicating that the defect produced by the H479Q mutation is related to ATP binding and positioning, and not to the ATPase catalytic mechanism. The importance of these findings is related to the presence of a corresponding amino acid in the human copper ATPase, whose mutation in Wilson disease produces analogous effects (36).
This work was supported, in whole or in part, by National Institutes of Health Grant NHLBI RO301–69830 (to G. I.). This work was also supported by a Special Promoted Project grant from the Ministry of Education, Culture, Sports, Science and Technology of Japan (to C. T.). The costs of publication of this article were defrayed in part by the payment of page charges. This article must therefore be hereby marked “advertisement” in accordance with 18 U.S.C. Section 1734 solely to indicate this fact.
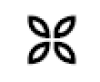
Author's Choice—Final version full access.
Footnotes
The abbreviations used are: NMBD, N-metal binding domain; MOPS, 3-(N-morpholino)propanesulfonic acid; WT, wild type; DDM, n-dodecyl-β-d-maltoside; BCS, bathocuproine disulfonate; MES, 4-morpholinethanesulfonic acid; DTT, dithiothrietol; ICP-MS, inductively coupled plasma mass spectrometry; SERCA, sarcoendoplasmic reticulum Ca2+ ATPase.
References
- 1.Pedersen, P. L., and Carafoli, E. (1987) Trends Biochem. Sci. 12 146-150 [Google Scholar]
- 2.Lutsenko, S., and Kaplan, J. H. (1995) Biochemistry 34 15607-15613 [DOI] [PubMed] [Google Scholar]
- 3.Moller, J. V., Juul, B., and Le Maire, M. (1996) Biochim. Biophys. Acta 1286 1-51 [DOI] [PubMed] [Google Scholar]
- 4.Axelsen, K. B., and Palmgern, M. G. (1998) J. Mol. Evol. 46 84-101 [DOI] [PubMed] [Google Scholar]
- 5.Arguello, J. M. (2003) J. Membr. Biol. 195 93-1008 [DOI] [PubMed] [Google Scholar]
- 6.Williams, L. E., Pittman, J. K., and Hall, J. L. (2000) Biochim. Biophys. Acta 1465 104-126 [DOI] [PubMed] [Google Scholar]
- 7.Silver, S. (1996) Gene (Amst.) 179 9-19 [DOI] [PubMed] [Google Scholar]
- 8.Bull, P. C., and Cox, D. W. (1994) Trends Genet. 10 246-252 [DOI] [PubMed] [Google Scholar]
- 9.Petris, M. J., Strausak, D., and Mercer, J. F. (2000) Hum. Mol. Genet. 9 2845-2851 [DOI] [PubMed] [Google Scholar]
- 10.Lutsenko, S., Barnes, N. L., Bartee, M. Y., and Dmitriev, O. Y. (2007) Physiol. Rev. 87 1011-1046 [DOI] [PubMed] [Google Scholar]
- 11.Petrukhin, K., Lutsenko, S., Chernov, I., Ross, B. M., Kaplan, J. H., and Gilliam, T. C. (1994) Hum. Mol. Genet. 3 1647-1656 [DOI] [PubMed] [Google Scholar]
- 12.Bull, P. C., Thomas, G. R., Rommens, J. M., Forbed, J. R., and Cox, D. W. (1993) Nat. Genet. 5 327-337 [DOI] [PubMed] [Google Scholar]
- 13.Vulpe, C., Levinson, B., Whitney, S., Packman, S., and Gitschier, J. (1993) Nat. Genet. 3 7-13 [DOI] [PubMed] [Google Scholar]
- 14.Solioz, M., and Camakaris, J. (1996) J. Biol. Chem. 271 24465-244708798705 [Google Scholar]
- 15.Solioz, M., and Odermatt, A. (1995) J. Biol. Chem. 270 9217-9221 [DOI] [PubMed] [Google Scholar]
- 16.Okkeri, J., and Haltia, T. (1999) Biochemistry 38 14109-14116 [DOI] [PubMed] [Google Scholar]
- 17.Sharma, R., Rensing, C., Rosen, B. P., and Mitra, B. (2000) J. Biol. Chem. 275 3873-3878 [DOI] [PubMed] [Google Scholar]
- 18.Rensing, C., Mitra, B., and Rosen, B. P. (1997) Proc. Natl. Acad. Sci. U. S. A. 94 14326-14331 [DOI] [PMC free article] [PubMed] [Google Scholar]
- 19.Tsai, K. J., Yoon, K. P., and Lynn, A. R. (1992) J. Bacteriol. 164 116-121 [DOI] [PMC free article] [PubMed] [Google Scholar]
- 20.MacLennan, D. H., Brandl, C. J., Korczak, B., and Green, N. M. (1985) Nature 316 696-700 [DOI] [PubMed] [Google Scholar]
- 21.Toyoshima, C., Nakasako, M., Nomura, H., and Ogawa, H. (2000) Nature 405 647-655 [DOI] [PubMed] [Google Scholar]
- 22.Dmitriev, O., Tsivkoskii, R., Abilgaard, F., Morgan, C. T., Markely, J. L., and Lutsenko, S. (2006) Proc. Natl. Acad. Sci. U. S. A. 103 5302-5307 [DOI] [PMC free article] [PubMed] [Google Scholar]
- 23.Sazinsky, M. H., Mandal, A. K., Arguello, J. M., and Rosenzweig, A. C. (2006) J. Biol. Chem. 281 11161-11166 [DOI] [PubMed] [Google Scholar]
- 24.Sazinsky, M. H., Agarwal, S., Arguello, J. M., and Rosenzweig, A. C. (2006) Biochemistry 45 9949-9955 [DOI] [PubMed] [Google Scholar]
- 25.Chintalapati, S., Al Kurdi, R., Terwisscha van Scheltinga, A. C., and Kühlbrandt, W. (2008) J. Mol. Biol. 378 581-595 [DOI] [PubMed] [Google Scholar]
- 26.Solioz, M., and Vulpe, C. (1996) Trends Biochem. Sci. 21 237-241 [PubMed] [Google Scholar]
- 27.Walker, J. M., Huster, D., Ralle, M., Morgan, C. T., Blackburn, N., and Lutsenko, S. (2004) J. Biol. Chem. 279 15376-15384 [DOI] [PubMed] [Google Scholar]
- 28.Cobine, P. A., George, G. N., Jones, C. E., Wickramasinghe, W. A., Solioz, M., and Dameron, C. T. (2002) Biochemistry 41 5822-5829 [DOI] [PubMed] [Google Scholar]
- 29.Malthaup, G., Strausak, D., Bissig, K. D., and Solioz, M. (2001) Biochem. Biophys. Res. Commun. 288 172-177 [DOI] [PubMed] [Google Scholar]
- 30.Tsivkovskii, R., MacArthur, B. C., and Lutsenko, S. (2001) J. Biol. Chem. 276 2234-2242 [DOI] [PubMed] [Google Scholar]
- 31.Mandal, A. K., Cheung, W. D., and Argüello, J. M. (2002) J. Biol. Chem. 277 7201-7208 [DOI] [PubMed] [Google Scholar]
- 32.Mandal, A. K., and Arguello, J. M. (2003) Biochemistry 42 11040-11047 [DOI] [PubMed] [Google Scholar]
- 33.Rice, W. J., Kovalishin, A., and Stokes, D. L. (2006) Biochem. Biophys. Res. Commun. 348 124-131 [DOI] [PubMed] [Google Scholar]
- 34.Nelson, K. E., Clayton, R. A., Gill, S. R., Gwinn, M. L., Dodson, R. J., Haft, D. H., Hickey, E. K., Peterson, J. D., Nelson, W. C., Ketchum, K. A., McDonald, L., Utterback, T. R., Malek, J. A., Linher, K. D., Garrett, M. M., Stewart, A. M., Cotton, M. D., Pratt, M. S., Phillips, C. A., Richardson, D., Heidelberg, J., Sutton, G. G., Fleischmann, R. D., Eisen, J. A., White, O., Salzberg, S. L., Smith, H. O., Venter, J. C., and Fraser, C. M. (1999) Nature 399 323-329 [DOI] [PubMed] [Google Scholar]
- 35.Hatori, Y., Majima, E., Tsuda, T., and Toyoshima, C. (2007) J. Biol. Chem. 282 25213-25221 [DOI] [PubMed] [Google Scholar]
- 36.Tsivkovskii, R., Efremov, G. R., and Lutzenko, S. (2003) J. Biol. Chem. 278 13302-13308 [DOI] [PubMed] [Google Scholar]
- 37.Eletr, S., and Inesi, G. (1972) Biochim. Biophys. Acta 282 174-179 [DOI] [PubMed] [Google Scholar]
- 38.Lanzetta, P. A., Alvarez, L. J., Reinsch, P. S., and Candia, O. A. (1979) Anal. Biochem. 100 95-97 [DOI] [PubMed] [Google Scholar]
- 39.Weber, K., and Osborn, M. (1969) J. Biol. Chem. 244 4406-4412 [PubMed] [Google Scholar]
- 40.de Meis, L., and CostaCarvalho, M. (1974) Biochemistry 13 5032-5038 [DOI] [PubMed] [Google Scholar]
- 41.Inesi, G., Goodman, J., and Watanabe, S. (1967) J. Biol. Chem. 242 4637-4643 [PubMed] [Google Scholar]
- 42.Jensen, A. M., Sørensen, T. L., Olesen, C., Møller, J. V., and Nissen, P. (2006) EMBO J. 25 2305-2314 [DOI] [PMC free article] [PubMed] [Google Scholar]
- 43.Masuda, H., and deMeis, L. (1973) Biochemistry 12 4581-4585 [DOI] [PubMed] [Google Scholar]
- 44.Gonzales-Guerrero, M., and Arguello, J. M. (2008) Proc. Natl. Acad. Sci. U. S. A. 105 5992-5997 [DOI] [PMC free article] [PubMed] [Google Scholar]
- 45.Burroughs, A. M., Allen, K. N., Dunaway-Mariano, D., and Aravind, L. (2006) J. Mol. Biol. 361 1003-1034 [DOI] [PubMed] [Google Scholar]
- 46.Cho, H., Wang, W., Kim, R., Yokota, H., Damo, S., Kim, S. H., Wemmer, D., Kustu, S., and Yan, D. (2001) Proc. Natl. Acad. Sci. U. S. A. 98 8525-8530 [DOI] [PMC free article] [PubMed] [Google Scholar]
- 47.Lubben, M., Guldenhaupt, J., Zoltner, M., Deigweiher, K., Haebel, P., Urbanke, C., and Scheidig, A. J. (2007) J. Mol. Biol. 369 363-368 [DOI] [PubMed] [Google Scholar]
- 48.Toyoshima, C., and Inesi, G. (2004) Annu. Rev. Biochem. 73 269-292 [DOI] [PubMed] [Google Scholar]
- 49.Inesi, G., Lewis, D., Toyoshima, C., Hirata, A., and de Meis, L. (2008) J. Biol. Chem. 283 1189-1196 [DOI] [PubMed] [Google Scholar]