Abstract
GABAB receptors are the G-protein-coupled receptors for γ-aminobutyric acid (GABA), the main inhibitory neurotransmitter in the brain. GABAB receptors are promising drug targets for a wide spectrum of psychiatric and neurological disorders. Receptor subtypes exhibit no pharmacological differences and are based on the subunit isoforms GABAB1a and GABAB1b. GABAB1a differs from GABAB1b in its ectodomain by the presence of a pair of conserved protein binding motifs, the sushi domains (SDs). Previous work showed that selectively GABAB1a contributes to heteroreceptors at glutamatergic terminals, whereas both GABAB1a and GABAB1b contribute to autoreceptors at GABAergic terminals or to postsynaptic receptors. Here, we describe GABAB1j, a secreted GABAB1 isoform comprising the two SDs. We show that the two SDs, when expressed as a soluble protein, bind to neuronal membranes with low nanomolar affinity. Soluble SD protein, when added at nanomolar concentrations to dissociated hippocampal neurons or to acute hippocampal slices, impairs the inhibitory effect of GABAB heteroreceptors on evoked and spontaneous glutamate release. In contrast, soluble SD protein neither impairs the activity of GABAB autoreceptors nor impairs the activity of postsynaptic GABAB receptors. We propose that soluble SD protein scavenges an extracellular binding partner that retains GABAB1a-containing heteroreceptors in proximity of the presynaptic release machinery. Soluble GABAB1 isoforms like GABAB1j may therefore act as dominant-negative inhibitors of heteroreceptors and control the level of GABAB-mediated inhibition at glutamatergic terminals. Of importance for drug discovery, our data also demonstrate that it is possible to selectively impair GABAB heteroreceptors by targeting their SDs.
GABAB receptors mediate pre- and postsynaptic inhibition in the nervous system and are implicated in a variety of disorders, including cognitive impairments, anxiety, depression, and epilepsy (1-3). Presynaptic GABAB receptors prevent neurotransmitter release via inhibition of Ca2+ channels (4) and second messenger-mediated effects downstream of Ca2+ entry (5-8). They are commonly divided into auto- and heteroreceptors depending on whether they control the release of GABA3 or other neurotransmitters, respectively. Postsynaptic GABAB receptors activate Kir3-type K+ channels and generate slow inhibitory postsynaptic currents (IPSCs) that hyperpolarize the cell and shunt excitatory currents (9). Recombinant and native studies showed that functional GABAB receptors are obligate heteromers composed of GABAB1 and GABAB2 subunits (10-13). Molecular diversity in the GABAB receptor system arises from the expression of multiple GABAB1 subunit isoforms. The GABAB1a and GABAB1b isoforms constitute two independently regulated receptor subtypes, GABAB(1a,2) and GABAB(1b,2), whereas the functional role of several secreted GABAB1 isoforms remains unclear (1, 14-17). Structurally, the GABAB1a and GABAB1b isoforms solely differ in their N-terminal ectodomain by a tandem pair of SDs that are present in GABAB1a but not in GABAB1b (18, 19). SDs, also known as complement control modules or short consensus repeats, mediate protein interactions in adhesion molecules and in G-protein-coupled receptors binding to peptide hormones (20, 21). Pharmacological tools that distinguish GABAB(1a,2) and GABAB(1b,2) receptors are lacking; however, the native roles of GABAB1a and GABAB1b were dissociated using GABAB1a-/- (1a-/-) and GABAB1b-/- (1b-/-) mice, which express one or the other isoform (22). These mice revealed that heteroreceptors incorporate the GABAB1a subunit, whereas autoreceptors and postsynaptic GABAB receptors incorporate GABAB1a or GABAB1b subunits (22-24). This suggests that the SDs of GABAB1a bind to protein(s) that localize heteroreceptors at glutamatergic terminals. A protein binding to the first SD of GABAB1a is the extracellular matrix protein fibulin-2, but whether it mediates GABAB receptor localization is unknown (19).
In addition to the membrane-bound GABAB1a and GABAB1b subunit isoforms, the GABAB1 gene produces several secreted isoforms that all include the SDs (14-16). Secreted isoforms containing SDs were also described for other receptors and shown to exert dominant-negative effects by scavenging the binding partners of the membrane-bound receptor (25, 26). Here, we identified a novel secreted GABAB1 isoform containing the SDs and addressed whether such soluble isoforms have the potential to block neuronal GABAB receptor functions in a dominant-negative manner.
EXPERIMENTAL PROCEDURES
Characterization of GABAB1j cDNA and mRNA—An oligo(dT) primed double-stranded cDNA made from the cortex/cerebellum of 7-day-old rats (34) was screened with a 32P-labeled SD-specific cDNA hybridization probe as described (27). For Northern blot analysis, total RNA was isolated from mouse brain and cultured mouse cortical neurons using TRIzol reagent (Invitrogen). In situ hybridization was performed as described previously (28) using digoxigenin-labeled GABAB1j-specific antisense RNA probes.
GABAB1j Protein Expression—Because a GABAB1j-specific antibody is lacking, we tagged GABAB1a and GABAB1j with the c-Myc epitope (29) and inserted the cDNAs into the expression vector pCI (Promega). Conditioned medium of transfected HEK293 cells (Lipofectamine 2000, Invitrogen) was collected after 48 h and used to immunoprecipitate secreted GABAB1 protein. Briefly, the medium was incubated with protein G-agarose (Roche Applied Science) for 2 h, precleared by centrifugation at 10,000 × g for 10 min, and incubated overnight with a monoclonal anti-Myc antibody (9E10, Sigma-Aldrich, diluted 1:1000) coupled to protein G-agarose. After five washes in radio immunoprecipitation assay buffer (150 mm NaCl, 1% Nonidet P-40, 0.5% sodium deoxycholate containing a protease inhibitor mixture (Roche Applied Science)), immunoprecipitated proteins were eluted from the protein G-agarose using 2× SDS loading buffer, separated on SDS-PAGE, and analyzed by Western blotting. To control for GABAB1a and GABAB1j expression levels, transfected HEK293 cells were lysed in radio immunoprecipitation assay buffer, and the lysate was precleared at 10,000 × g for 10 min and mixed with 2× SDS loading buffer. For Western blot analysis, we used rabbit polyclonal anti-Myc (PRB-150C diluted 1:1000, Covance) and peroxidase-coupled secondary antibodies (donkey anti-rabbit diluted 1:2500, Amersham Biosciences). Blots were developed using the enhanced chemiluminescence detection system (Amersham Biosciences) and exposed to Kodak Bio-Max maximum resolution x-ray films (Sigma-Aldrich).
To detect native GABAB1j protein, we generated the anti-SD monoclonal antibody 43H12. GABAB1-deficient mice (11) were immunized intraperitoneally with 50 μg of GST·SD fusion protein in alum, and after 4 weeks, they were boosted intravenously with 10 μg of GST·SD fusion protein in phosphate-buffered saline. 5 days after boosting, spleen cells were used to generate hybridomas, which were screened for the production of IgG anti-SD antibodies (30). Prior to metabolic labeling, cortical neurons in culture (31) were incubated for 30 min in 15 ml of methionine- and cysteine-free Dulbecco's modified Eagle's medium (Sigma-Aldrich) containing 1% dialyzed fetal calf serum. The cells were then labeled for 5 h with 5 ml of 150 μCi/ml 35S-EXPRESS protein labeling mix (PerkinElmer Life Sciences). GABAB1 proteins with SDs were immunoprecipitated from conditioned cell culture medium and lysed cells (radio immunoprecipitation assay buffer) using anti-SD antibody 43H12. Radiolabeled proteins were revealed by autoradiography after SDS-PAGE.
125I-Tyr-RSDP Binding—Recombinant SD protein (RSDP) and mutant RSDP (mutRSDP) were produced and purified as described (supplemental materials). RSDP was dialyzed against 10 mm MES buffer (pH 6.1, Sigma-Aldrich), concentrated to 0.95 mg/ml by ultrafiltering (anisotropic membrane YM-10 Centricon, Millipore), and labeled with 125I to a specific activity of 1846 Ci/mmol (ANAWA Trading SA). To prepare membranes for competition binding experiments, CHO-K1 (ATCC) and 293FT (Invitrogen) cells were homogenized in Krebs-Tris buffer (20 mm Tris-Cl, pH 7.4, 118 mm NaCl, 5.6 mm glucose, 1.2 mm KH2PO4, 1.2 mm MgSO4, 4.7 mm KCl, 1.8 mm CaCl2) and centrifuged for 30 min at 40,000 × g, and the pellet was resuspended in buffer. Rat cortex synaptic membranes were prepared as described (27). Membranes were suspended in Krebs-Tris buffer supplemented with 0.2% (w/v) bovine serum albumin at a concentration of 200-400 μg/ml. 100-μl aliquots were incubated with 0.5 nm 125I-Tyr-RSDP for 90 min at room temperature in the presence or absence of unlabeled RSDP protein. After cooling on ice for 20 min, samples were centrifuged for 30 min at 20,000 × g (4 °C). The pellet was rinsed three times with 1 ml of ice-cold buffer, and the radioactivity was determined by Cerenkov counting. Concentration response curves were generated from triplicate determinations (GraphPad).
Electrophysiology—300-μm-thick horizontal hippocampal slices were prepared from postnatal day 22-28 mice (VT 1000 vibratome, Leica) in cooled artificial cerebro-spinal fluid (ACSF) (in mm: 119 NaCl, 2.5 KCl, 1.3 MgCl2, 2.5 CaCl2, 1.0 NaH2PO4, 26.2 NaHCO3, and 11 glucose) equilibrated with 95% O2, 5% CO2 at pH 7.3. After recovery for >1 h, slices were incubated for 6 h with RSDP (1.0 μg/ml), transferred to the recording chamber, and superfused (2 ml/min) with ACSF at 30-32 °C. Visualized whole cell voltage clamp recording was used to measure holding currents (Kir3 channels) and synaptic currents from the somata of CA1 pyramidal neurons. Synaptic currents were evoked by voltage pulses (100 μs, 2-5 V) delivered through a bipolar Pt-Ir electrode (25 μm in diameter) placed in the stratum radiatum. Miniature postsynaptic currents were recorded in the presence of tetrodotoxin (0.5 μm, Latoxan). For measuring miniature and evoked currents, patch electrodes (∼3 megaohms) were filled with a solution containing, in mm: 30 cesium gluconate, 100 CsCl, 4 MgCl2, 10 creatine phosphate, 3.4 Na2ATP, 0.1 Na3GTP, 1.1 EGTA, and 5 Hepes (pH adjusted to 7.3 with KOH). Adenosine-mediated presynaptic inhibition was measured in the presence of CGP54626 (2 μm). For measuring Kir3 currents, cesium gluconate and CsCl were replaced by 130 mm potassium gluconate. GABAergic and glutamatergic currents were pharmacologically isolated using kynurenic acid (2 mm) and picrotoxin (100 μm), respectively. Neurons were clamped at -50 mV. Currents were amplified (Axopatch 200B, Axon Instruments), filtered at 1 kHz, and digitized at 5 kHz. Miniature excitatory postsynaptic currents (mEPSCs) and miniature inhibitory postsynaptic currents (mIPSCs) were detected and analyzed using MiniAnalysis software (version 6.0.3, Synaptosoft, Decatur, GA). Significant differences between two distributions of mEPSC and mIPSC amplitude and interevent intervals were determined by using the Kolmogorov-Smirnov test, with p < 0.01 indicating significance. Evoked synaptic currents were analyzed using analysis of variance (GraphPad), with p < 0.01 indicating significance. Kir3 currents were statistically analyzed using the nonparametric Mann-Whitney test, with p ≤ 0.05 indicating significance (INSTAT, version 3.0, GraphPad). The recording of miniature postsynaptic currents in dissociated cultured neurons was as described above. Hippocampal neurons were prepared from 16.5-day mouse embryos and cultured at a density of ∼750 cells/mm2 on poly-l-lysine coated glass coverslips for 3 weeks, as described (31). Neurons with a basal frequency of ∼3 Hz were used in the experiments. The experimenter was blind to the treatment of the hippocampal cultures or the slices. Baclofen and CGP54626 were from Novartis, and all other reagents were from Fluka or Sigma-Aldrich.
RESULTS
GABAB1j Encodes a Secreted Glycoprotein—To identify GABAB1 isoforms, we screened a rat cortex/cerebellum cDNA library with an SD-specific hybridization probe. We isolated cDNAs for a novel isoform of ∼1.6 kb that we named GABAB1j. GABAB1j diverges from GABAB1a downstream of exon 4 and encodes a protein of 229 amino acids (Fig. 1A and supplemental Fig. 1A). The N-terminal 157 amino acids of GABAB1j are identical to GABAB1a and encode the signal peptide as well as the two SDs; the C-terminal 72 residues exhibit no significant homology to known proteins. Northern blot analysis revealed GABAB1j transcripts of ∼1.6 kb in brain tissue and cultured cortical neurons (Fig. 1B). An SD-specific hybridization probe demonstrated that GABAB1j and GABAB1a transcripts are of similar abundance (Fig. 1B). The GABAB1j transcript distribution in brain sections (Fig. 1C) is similar to that described for GABAB1a (32). Hydropathicity analysis revealed that GABAB1j protein lacks transmembrane domains (supplemental Fig. 1B). Western blot analysis of transiently transfected HEK293 cells showed that the Myc-tagged GABAB1j protein has a molecular mass of ∼29 kDa (Fig. 1D). Deglycosylation of GABAB1j with peptide N-glycosidase F decreased the molecular mass to ∼23 kDa (data not shown), which corresponds to the calculated molecular weight of the mature protein. Immunoprecipitation experiments recovered GABAB1j but not membrane-bound GABAB1a from conditioned HEK293 cell-culture medium, demonstrating that GABAB1j is a secreted protein (Fig. 1D). We next addressed whether endogenously expressed GABAB1j protein is detectable in neurons. We did not succeed in generating a GABAB1j-specific antibody; however, we generated an anti-SD monoclonal antibody that immunoprecipitates GABAB1a protein from brain tissue (Fig. 1E, left panel). In addition, the antibody immunoprecipitates a protein with a molecular mass corresponding to that of GABAB1j from metabolically labeled cortical neurons (Fig. 1E, right panel). In the absence of a specific GABAB1j antibody, this provides indirect evidence for the existence of a stable GABAB1j protein in vivo.
FIGURE 1.
Characterization of the GABAB1j isoform. A, schematic representation of the 5′ end of the GABAB1 gene indicating the exons encoding the GABAB1a, GABAB1b, and GABAB1j isoforms. GABAB1j results from an 870-bp extension of exon 4 at its 3′ end (exon 4′), generating an open reading frame of 687 nucleotides encompassing the two SDs. B, Northern blot analysis of GABAB1a and GABAB1j transcripts. Total RNA extracted from primary mouse cortical (ctx) neurons in culture or mouse brain was hybridized to the 32P-labeled probes indicated in A. The pan probe encodes part of the extracellular GABA binding domain and detects ∼4.5-kb GABAB1a and ∼4.1-kb GABAB1b transcripts (not resolved). The SD1/2 probe encodes the two SDs and detects GABAB1a and ∼1.6-kb GABAB1j transcripts. The 1j probe encodes 510 nucleotides at the 3′ end of exon 4′. C, in situ hybridization with the digoxigenin-labeled 1j probe. Top, horizontal section depicting the dorsal tier of the brain; bottom, high magnification of coronal section depicting lobules of the cerebellum. The locations of the CA1/3 field of hippocampus proper (CA1/3), dentate gyrus (DG), medial habenula (MH), and the granular layer (GL) and molecular layer (ML) of the cerebellum are indicated. Scale bars, 2 mm (top) and 200 μm (bottom). D, HEK293 cells expressing Myc-tagged GABAB1a (myc-1a) or GABAB1j (myc-1j) proteins. Conditioned medium (cond. med.) was subjected to immunoprecipitation with a rabbit anti-Myc antibody and analyzed in parallel with total cell lysate on Western blots using a mouse anti-Myc antibody. Membrane-bound GABAB1a protein was selectively detected in the cell lysate, whereas secreted GABAB1j protein was additionally detected in the cell-conditioned medium. E, left panel, the anti-SD monoclonal antibody 43H12 immunoprecipitates GABAB1a but not GABAB1b from mouse brain lysates. Immunoprecipitated GABAB1 protein (IP anti-SD) was analyzed in parallel with total brain lysate (input) on Western blots using a pan GABAB1 antibody (12). Right panel, the anti-SD monoclonal antibody 43H12 immunoprecipitates two proteins with a molecular mass corresponding to that of GABAB1a (**) and GABAB1j (*) from metabolically labeled cortical neurons. Radiolabeled proteins were revealed by autoradiography.
Neuronal Membranes Exhibit High Affinity Binding Sites for the SDs—To address whether SDs interact with specific binding sites in neuronal membranes, we produced a truncated GABAB1j protein containing the two SDs but lacking the C-terminal 72 residues (Fig. 2A). This RSDP was radiolabeled with 125I at tyrosine residues and used in competition binding experiments. Approximately half of the 125I-Tyr-RSDP bound to rat cortex synaptic membranes was specifically displaced by unlabeled RSDP (Fig. 2B). No specific 125I-Tyr-RSDP binding sites were detected in cell membranes of HEK293FT and CHO-K1 cells (Fig. 2B). Concentration-response curves revealed a half-maximal inhibition of 125I-Tyr-RSDP binding at ∼2 nm of unlabeled RSDP (n = 3, 95% confidence interval 1.1-3.4 nm; Fig. 2C).
FIGURE 2.
Specific binding sites for 125I-Tyr-RSDP in rat cortex synaptic membranes. A, expression of RSDP in Pichia pastoris. Top, a schematic representation of RSDP containing the two SDs flanked by two tobacco etch virus cleavage sites (TEVcs) and C-terminal c-Myc and polyhistidine (Hisx6) tags. Bottom, recombinant protein identified on Western blots using anti-His6 antibodies. RSDP is N-glycosylated as indicated by the shift from ∼29 kDa to the calculated molecular mass of ∼23 kDa after peptide N-glycosidase F (PNGaseF) treatment. RSDP is stable at 37 °C for at least 7 days (data not shown). B, 125I-Tyr-RSDP (0.5 nm) binding to 20 μg of membranes from cortex, CHO-K1, and HEK293FT cells, in the absence or presence of 200 nm unlabeled RSDP protein. Data are means ± S.D. from three independent experiments. C, inhibition of 125I-Tyr-RSDP (0.5 nm) binding to 40 μg of cortical membranes by different concentrations of unlabeled RSDP. The inhibition curve was calculated using nonlinear regression. Data points are means ± S.E. from three independent experiments.
RSDP Impairs GABAB Receptor-mediated Inhibition of Spontaneous Glutamate Release—GABAB heteroreceptors inhibit the spontaneous release of glutamate, likely by interfering with the release process downstream of Ca2+ entry (7, 8, 22). We addressed whether exogenous application of RSDP to dissociated hippocampal neurons in culture exerts a dominant-negative effect on heteroreceptors by scavenging a binding partner of their GABAB1a subunits. Under control conditions in ACSF, the GABAB receptor agonist baclofen (100 μm) significantly reduced the frequency (Fig. 3, A and B) but not the amplitude (data not shown) of mEPSCs recorded from pyramidal neurons, consistent with a presynaptic mode of action. At 4 nm of RSDP, a maximal impairment is seen after 12 h (Fig. 3A). At 40 nm of RSDP, a partial impairment of presynaptic inhibition was observed as early as 10 min after RSDP application, whereas a near complete impairment was observed after 1 h (Fig. 3, A and B). This shows that the effect of RSDP is concentration-dependent. RSDP did not interfere with the inhibition of spontaneous glutamate release mediated by adenosine A1 receptors (Fig. 3, C and D), which converge on the same effectors as GABAB receptors (7, 22). This demonstrates that RSDP does not indiscriminately act at presynaptic G-protein-coupled receptors.
FIGURE 3.
RSDP impairs GABAB receptor-mediated inhibition of spontaneous glutamate release in dissociated hippocampal neurons in culture. A, the percentage of inhibition of the mEPSC frequency by baclofen (100 μm) was assessed in individual neurons under control condition (ACSF, n = 5) and after incubation with 4 nm (n = 5) or 40 nm (n = 7) RSDP for the times indicated (for values, see supplemental Table S1). B, time course of the RSDP effect on the baclofen-induced mEPSC frequency inhibition in individual neurons (n = 5 per condition). C, summary histograms illustrating that incubation with 40 nm RSDP for 1 h impairs baclofen (bac)-but not adenosine (adeno)-mediated mEPSC frequency inhibition. Values are means ± S.E. of the percentage of inhibition of the mEPSC frequency (100 μm baclofen, ACSF, 88.6 ± 2.4% n = 5; RSDP, 15.7 ± 3.2%, n = 5, ***, p < 0.001, Kolmogorov-Smirnov; 100 μm adenosine, ACSF, 70.2 ± 4.0%, n = 5; RSDP, 78.6 ± 2.7%, n = 5). D, representative mEPSC recordings under baseline conditions, during adenosine application, after washing with ACSF (wash), during baclofen application, and after antagonizing GABAB receptors with CGP54626. Recordings from one cell each incubated with ACSF or RSDP are shown.
RSDP Selectively Impairs GABAB(1a,2) Receptors Located at Glutamatergic Terminals—We next investigated whether RSDP similarly impairs GABAB heteroreceptors in acute hippocampal slices. Under control conditions, baclofen significantly reduced the mEPSC frequency recorded from CA1 pyramidal neurons (Fig. 4A), whereas there was no significant change in the mEPSC amplitude distribution (not shown). Consistent with the results obtained with dissociated neurons, baclofen was ineffective in reducing the mEPSC frequency after incubation of slices with 40 nm RSDP (Fig. 4A). Baclofen is also described to inhibit the mIPSC frequency by acting at autoreceptors (5, 33). Although baclofen inhibited the frequency of mIPSC recorded from CA1 pyramidal neurons, RSDP was without effect on this inhibition (Fig. 4A). Likewise, RSDP did not alter the amplitude of Kir3 currents induced by baclofen in CA1 pyramidal neurons (9, 11, 22), demonstrating that RSDP has no effect on postsynaptic GABAB receptors (Fig. 4A). These data suggest that RSDP selectively interferes with the function of GABAB heteroreceptors, which incorporate the GABAB1a subunit. However, GABAB1a also contributes to autoreceptors and postsynaptic GABAB receptors (22-24). In the above experiments, RSDP effects on GABAB(1a,2) autoreceptors or postsynaptic GABAB(1a,2) receptors may remain undetected due to the concomitant action of GABAB(1b,2) receptors at GABAergic terminals and postsynaptic sites. We therefore used hippocampal slices of 1b-/- mice to address whether RSDP interferes with the activity of GABAB(1a,2) autoreceptors and postsynaptic GABAB(1a,2) receptors. We found that in 1b-/- slices, RSDP neither impaired autoreceptor responses nor impaired baclofen-activated Kir3 currents (Fig. 4B). In contrast, RSDP strongly impaired heteroreceptor responses in 1b-/- slices (Fig. 4B), thus corroborating the data obtained in wild-type slices (Fig. 4A). RSDP was without effect on pre- and postsynaptic GABAB responses in 1a-/- mice, in which all GABAB receptors incorporate the GABAB1b subunit (Fig. 4C). As a control, RSDP again failed to impair the actions of pre- and postsynaptic adenosine A1 receptors in all genotypes (Fig. 4, A-C). In summary, these results demonstrate that RSDP exclusively impairs GABAB(1a,2) receptors located at glutamatergic terminals.
FIGURE 4.
RSDP selectively impairs GABAB(1a,2) receptors located at glutamatergic terminals. A, the percentage of mEPSC and mIPSC frequency inhibition by baclofen (bac, 100 μm) and adenosine (adeno, 100 μm) under control condition (ACSF, white bars) and after incubation of acute hippocampal slices from wild-type (WT) mice with RSDP (40 nm, black bars) for 6 h. Baclofen was significantly less efficient in reducing the frequency of mEPSCs recorded from CA1 pyramidal neurons incubated with RSDP than from neurons incubated with ACSF (ACSF, 71.0 ± 5.4% inhibition, n = 5; RSDP, 7.6 ± 2.2% inhibition, n = 9; ***, p < 0.001, Kolmogorov-Smirnov; see also supplemental Table S2). RSDP was without effect on adenosine-mediated mEPSC frequency inhibition (ACSF, 67.0 ± 5.3% inhibition, n = 5; RSDP, 76.0 ± 3.0% inhibition, n = 9; supplemental Table S2). As an additional control, RSDP was also without effect on the baseline mEPSC frequency or amplitude (for values, see supplemental Table S3). RSDP did not affect the baclofen- or adenosinemediated inhibition of the mIPSCs frequency recorded from CA1 pyramidal neurons (for values, see supplemental Table S2). RSDP did not alter the amplitudes of Kir3 current responses induced by baclofen and adenosine in CA1 pyramidal neurons (n = 6 per condition). B, RSDP impairs the baclofen-mediated mEPSC frequency inhibition in 1b-/- mice (ACSF, 67.0 ± 3.0% inhibition; RSDP, 6.0 ± 2.0%; n = 4; ***, p < 0.001, Kolmogorov-Smirnov). Incubation with RSDP had no effect on baclofen- or adenosine-mediated effects on the mIPSC frequency (for values, see supplemental Table S2) or on Kir3 current amplitudes. Baclofen-induced Kir3 current responses were reduced in the 1b-/- when compared with wild-type mice, as described (22). C, baclofen failed to depress the mEPSCs frequency in 1a-/- mice due to the lack of GABAB heteroreceptors in these mice (22). The basal mEPSC frequency in 1a-/- mice was increased (for values, see supplemental Table S3), as reported previously (22). RSDP was without effect on the baclofen- or adenosine-mediated inhibition of the mIPSC frequency (for values, see supplemental Table S2) or on Kir3 currents in 1a-/- mice. All values are means ± S.E.
RSDP Impairs GABAB Receptor-mediated Inhibition of Evoked Glutamate Release—Activation of presynaptic GABAB receptors not only reduces the frequencies of mEPSCs and mIPSCs but also reduces the amplitudes of evoked EPSCs and IPSCs (7, 11, 22). The inhibitory effect of baclofen on spontaneous release is believed to be mechanistically distinct from its effect on evoked release (5, 7, 8, 34). It therefore was interesting to address whether the effect of RSDP on the control of spontaneous glutamate release generalizes to the evoked release. Specifically, we tested whether RSDP interferes with the baclofen-induced reduction in the amplitudes of evoked EPSCs recorded from CA1 pyramidal neurons (22). Incubation of acute hippocampal slices with 40 nm RSDP essentially abolished the reduction of the EPSC amplitudes by baclofen (Fig. 5, A and B). In contrast, RSDP did not influence the baclofen-induced reduction of IPSC amplitudes recorded from CA1 pyramidal neurons (Fig. 5, A and B). As a control, RSDP failed to interfere with the reduction of evoked EPSC and IPSC amplitudes mediated by adenosine A1 receptors. These data confirm that RSDP specifically acts at GABAB heteroreceptors and show that RSDP affects spontaneous as well as evoked glutamate release (Fig. 5, A and B).
FIGURE 5.
RSDP selectively impairs the baclofen-induced decrease of evoked EPSC amplitudes in acute hippocampal slices. A, average traces (n = 100 events) depicting evoked EPSCs and IPSCs in CA1 pyramidal neurons before (black) and after inhibition (red) with baclofen (100 μm) or adenosine (100 μm). Recordings were after a 6-h incubation of slices with ACSF, RSDP (40 nm), reduced RSDP (treatment with 20 mm dithiothreitol for 2 h prior to slice application), and RSDP with mutated disulfide bridges (mutRSDP). B, summary histograms of the inhibition of EPSCs and IPSCs by baclofen (100 μm) and adenosine (100 μm) in CA1 pyramidal neurons. In the presence of RSDP, baclofen was less efficient in reducing EPSC amplitudes (ACSF, 85 ± 4% inhibition, n = 6; RSDP, 12.5 ± 3.5% inhibition, n = 6; ***, p < 0.001, analysis of variance). In contrast, reduced RSDP or mutRSDP were without effect on the baclofen-induced decrease of EPSC amplitudes. All treatment conditions did not impair the adenosine-mediated decrease in EPSC amplitudes (ACSF, 83 ± 2% inhibition, n = 6; RSDP, 85 ± 3% inhibition, n = 6). RSDP did not impair the baclofen- or adenosine-mediated inhibition of evoked IPSCs. Values are means ± S.E.
The tertiary structure of the SDs, which is fixed by two conserved intramolecular disulfide bridges, is critical for function (20). We produced a mutRSDP with serine substitutions of the first and fourth cysteine in each SD, which precludes disulfide bond formation (supplemental Fig. 2). We found that incubation of slices with mutRSDP or RSDP that was kept in a reduced state (treatment with 20 mm dithiothreitol) was without effect on the baclofen-induced reduction of evoked EPSC amplitudes, supporting that the binding function of RSDP is important for inhibiting heteroreceptors (Fig. 5A). Incubation of slices with 40 nm recombinant fibulin-2, a known binding partner of GABAB1a (19), did not interfere with the baclofen-induced inhibition of evoked glutamate release (supplemental Fig. 3, A and B). Moreover, 40 nm fibulin-2 did not neutralize the inhibitory action of 40 nm RSDP at heteroreceptors (supplemental Fig. 3C). It therefore appears that RSDP does not impair heteroreceptors by scavenging fibulin-2.
DISCUSSION
In this study, we describe GABAB1j, a secreted GABAB1 subunit isoform. GABAB1j, like all secreted GABAB1 isoforms (14-16), contains the SDs present in GABAB1a. Naturally occurring soluble SDs of other membrane-bound receptors were shown to exert physiologically relevant dominant-negative effects (25, 26). We therefore asked whether the SDs of secreted GABAB1 isoforms could act similarly and scavenge a putative extracellular binding partner of the membrane-bound GABAB1a subunit. Consistent with this proposal, we found that RSDP, a recombinant protein consisting of the two SDs, binds with low nanomolar affinity to specific binding sites in neuronal membranes. We also found that RSDP interferes with the activity of GABAB(1a,2) heteroreceptors, whereas having no effect at GABA(B1a,2) autoreceptors or postsynaptic GABA(B1a,2) receptors. These results imply that functionally relevant SD binding sites exist at the cell surface of glutamatergic terminals. In this context, it is interesting to note that other neurotransmitter receptors were recently shown to bind to extracellular partners that regulate their synaptic localization and functions (35-37). In our experiments, the extracellular matrix protein fibulin-2, which binds to the first SD of GABAB1a (19), was without effect on heteroreceptor function. We did not observe that RSDP co-immunoprecipitates with GABAB1a after co-expression in HEK293 cells, suggesting that SDs do not recruit heteroreceptors through homophilic interactions either (data not shown). Therefore, the auxiliary factor binding to SDs of GABAB1a at the cell surface remains to be identified.
How are GABAB heteroreceptors inactivated following RSDP exposure? First of all, we exclude that RSDP acts as a competitive antagonist of GABAB receptors because RSDP did not inhibit GABAB(1a,2)-mediated Kir3 responses in HEK293 cells (data not shown), nor did it inhibit the function of GABA(B1a,2) autoreceptors or postsynaptic GABA(B1a,2) receptors in hippocampal slices (Fig. 4B). It also appears unlikely that GABA(B1a,2) receptors rapidly internalize as a consequence of disrupting an extracellular interaction since neuronal GABAB receptors do not efficiently internalize (38). Moreover, GABA(B1a,2) autoreceptors or postsynaptic GABA(B1a,2) receptors are not affected by RSDP, suggesting that GABA(B1a,2) receptors are functional in the absence of an SD interaction (Fig. 4B). Since heteroreceptor impairment is seen within minutes of RSDP application to dissociated hippocampal neurons in culture, we also consider it unlikely that RSDP interferes with the axonal delivery of GABA(B1a,2) receptors. Most likely, the extracellular binding partner of the SDs acts as a diffusion trap that keeps heteroreceptors and elements of the release machinery in close proximity. RSDP may scavenge the SD binding partner and thereby promote lateral diffusion of heteroreceptors. This may explain why RSDP concomitantly interferes with GABAB effectors involved in the inhibition of spontaneous and evoked release.
Our data show that secreted GABAB1 isoforms like GABAB1j could, in principle, adjust the level of presynaptic inhibition at glutamatergic terminals. It therefore will be interesting to address whether the production of the various secreted GABAB1 isoforms is regulated in response to physiological stimuli. Of importance, our findings may also be exploited therapeutically. Drug development in the GABAB field was largely hampered because receptor subtypes cannot be distinguished pharmacologically. For example, it would be desirable to selectively inhibit heteroreceptors to boost excitatory neurotransmission in patients with cognitive impairments (39). Our experiments now directly show that this is possible by targeting the SDs.
Supplementary Material
Acknowledgments
We thank R. Seddik, H. R. Brenner, and K. Vogt for critical reading of the manuscript.
The nucleotide sequence(s) reported in this paper has been submitted to the GenBank™/EBI Data Bank with accession number(s) AM418837.
This work was supported by a grant from the European Community's Seventh Framework Programme (FP7/2007-2013) under Grant Agreement 201714. This study was also supported by the Swiss Science Foundation (Grant 3100-067100.01) and a grant from Novartis Pharma AG. The costs of publication of this article were defrayed in part by the payment of page charges. This article must therefore be hereby marked “advertisement” in accordance with 18 U.S.C. Section 1734 solely to indicate this fact.
The on-line version of this article (available at http://www.jbc.org) contains supplemental text, three supplemental figures, and three supplemental tables.
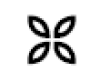
Author's Choice—Final version full access.
Footnotes
The abbreviations used are: GABA, γ-aminobutyric acid; EPSC, excitatory postsynaptic current; mEPSC, miniature EPSC; IPSC, inhibitory postsynaptic current; mIPSC, miniature IPSC; SD, sushi domain; RSDP, recombinant SD protein; mutRSDP, mutant RSDP; ACSF, artificial cerebrospinal fluid; MES, 4-morpholineethanesulfonic acid; CHO, Chinese hamster ovary.
References
- 1.Bettler, B., Kaupmann, K., Mosbacher, J., and Gassmann, M. (2004) Physiol. Rev. 84 835-867 [DOI] [PubMed] [Google Scholar]
- 2.Bowery, N. G., Bettler, B., Froestl, W., Gallagher, J. P., Marshall, F., Raiteri, M., Bonner, T. I., and Enna, S. J. (2002) Pharmacol. Rev. 54 247-264 [DOI] [PubMed] [Google Scholar]
- 3.Couve, A., Moss, S. J., and Pangalos, M. N. (2000) Mol. Cell. Neurosci. 16 296-312 [DOI] [PubMed] [Google Scholar]
- 4.Dunlap, K., and Fischbach, G. D. (1981) J. Physiol. (Lond.) 317 519-535 [DOI] [PMC free article] [PubMed] [Google Scholar]
- 5.Jarolimek, W., and Misgeld, U. (1997) J. Neurosci. 17 1025-1032 [DOI] [PMC free article] [PubMed] [Google Scholar]
- 6.Sakaba, T., and Neher, E. (2003) Nature 424 775-778 [DOI] [PubMed] [Google Scholar]
- 7.Scanziani, M., Capogna, M., Gahwiler, B. H., and Thompson, S. M. (1992) Neuron 9 919-927 [DOI] [PubMed] [Google Scholar]
- 8.Yamada, J., Saitow, F., Satake, S., Kiyohara, T., and Konishi, S. (1999) Neuropharmacology 38 1743-1753 [DOI] [PubMed] [Google Scholar]
- 9.Lüscher, C., Jan, L. Y., Stoffel, M., Malenka, R. C., and Nicoll, R. A. (1997) Neuron 19 687-695 [DOI] [PubMed] [Google Scholar]
- 10.Marshall, F. H., Jones, K. A., Kaupmann, K., and Bettler, B. (1999) Trends Pharmacol. Sci. 20 396-399 [DOI] [PubMed] [Google Scholar]
- 11.Schuler, V., Lüscher, C., Blanchet, C., Klix, N., Sansig, G., Klebs, K., Schmutz, M., Heid, J., Gentry, C., Urban, L., Fox, A., Spooren, W., Jaton, A. L., Vigouret, J. M., Pozza, M., Kelly, P. H., Mosbacher, J., Froestl, W., Käslin, E., Korn, R., Bischoff, S., Kaupmann, K., van der Putten, H., and Bettler, B. (2001) Neuron 31 47-58 [DOI] [PubMed] [Google Scholar]
- 12.Gassmann, M., Shaban, H., Vigot, R., Sansig, G., Haller, C., Barbieri, S., Humeau, Y., Schuler, V., Muller, M., Kinzel, B., Klebs, K., Schmutz, M., Froestl, W., Heid, J., Kelly, P. H., Gentry, C., Jaton, A. L., Van der Putten, H., Mombereau, C., Lecourtier, L., Mosbacher, J., Cryan, J. F., Fritschy, J. M., Luthi, A., Kaupmann, K., and Bettler, B. (2004) J. Neurosci. 24 6086-6097 [DOI] [PMC free article] [PubMed] [Google Scholar]
- 13.Prosser, H. M., Gill, C. H., Hirst, W. D., Grau, E., Robbins, M., Calver, A., Soffin, E. M., Farmer, C. E., Lanneau, C., Gray, J., Schenck, E., Warmerdam, B. S., Clapham, C., Reavill, C., Rogers, D. C., Stean, T., Upton, N., Humphreys, K., Randall, A., Geppert, M., Davies, C. H., and Pangalos, M. N. (2001) Mol. Cell. Neurosci. 17 1059-1070 [DOI] [PubMed] [Google Scholar]
- 14.Holter, J., Davies, J., Leresche, N., Crunelli, V., and Carter, D. A. (2005) J. Mol. Neurosci. 26 99-108 [DOI] [PubMed] [Google Scholar]
- 15.Schwarz, D. A., Barry, G., Eliasof, S. D., Petroski, R. E., Conlon, P. J., and Maki, R. A. (2000) J. Biol. Chem. 275 32174-32181 [DOI] [PubMed] [Google Scholar]
- 16.Wei, K., Jia, Z., Wang, Y. T., Yang, J., Liu, C. C., and Snead, O. C., III (2001) Brain Res. Mol. Brain Res. 89 103-110 [DOI] [PubMed] [Google Scholar]
- 17.Steiger, J. L., Bandyopadhyay, S., Farb, D. H., and Russek, S. J. (2004) J. Neurosci. 24 6115-6126 [DOI] [PMC free article] [PubMed] [Google Scholar]
- 18.Hawrot, E., Xiao, Y., Shi, Q. L., Norman, D., Kirkitadze, M., and Barlow, P. N. (1998) FEBS Lett. 432 103-108 [DOI] [PubMed] [Google Scholar]
- 19.Blein, S., Ginham, R., Uhrin, D., Smith, B. O., Soares, D. C., Veltel, S., McIlhinney, R. A., White, J. H., and Barlow, P. N. (2004) J. Biol. Chem. 279 48292-48306 [DOI] [PubMed] [Google Scholar]
- 20.Soares, D. C., and Barlow, P. N. (2005) in Structural Biology of the Complement System (Morikis, D., and Lambris, J. D., eds) pp. 19-62, CRC Press, Taylor and Francis Group, Boca Raton
- 21.Grace, C. R., Perrin, M. H., DiGruccio, M. R., Miller, C. L., Rivier, J. E., Vale, W. W., and Riek, R. (2004) Proc. Natl. Acad. Sci. U. S. A. 101 12836-12841 [DOI] [PMC free article] [PubMed] [Google Scholar]
- 22.Vigot, R., Barbieri, S., Brauner-Osborne, H., Turecek, R., Shigemoto, R., Zhang, Y. P., Lujan, R., Jacobson, L. H., Biermann, B., Fritschy, J. M., Vacher, C. M., Muller, M., Sansig, G., Guetg, N., Cryan, J. F., Kaupmann, K., Gassmann, M., Oertner, T. G., and Bettler, B. (2006) Neuron 50 589-601 [DOI] [PMC free article] [PubMed] [Google Scholar]
- 23.Shaban, H., Humeau, Y., Herry, C., Cassasus, G., Shigemoto, R., Ciocchi, S., Barbieri, S., van der Putten, H., Kaupmann, K., Bettler, B., and Luthi, A. (2006) Nat. Neurosci. 9 1028-1035 [DOI] [PubMed] [Google Scholar]
- 24.Ulrich, D., and Bettler, B. (2007) Curr. Opin. Neurobiol. 17 298-303 [DOI] [PubMed] [Google Scholar]
- 25.Bulanova, E., Budagian, V., Duitman, E., Orinska, Z., Krause, H., Ruckert, R., Reiling, N., and Bulfone-Paus, S. (2007) J. Biol. Chem. 282 13167-13179 [DOI] [PubMed] [Google Scholar]
- 26.Mosley, B., Beckmann, M. P., March, C. J., Idzerda, R. L., Gimpel, S. D., VandenBos, T., Friend, D., Alpert, A., Anderson, D., Jackson, J., Wignall, J. M., Smith, C., Gallis, B., Sims, J. E., Urdal, D., Widmer, M. B., Cosman, D., and Park, L. (1989) Cell 59 335-348 [DOI] [PubMed] [Google Scholar]
- 27.Kaupmann, K., Huggel, K., Heid, J., Flor, P. J., Bischoff, S., Mickel, S. J., McMaster, G., Angst, C., Bittiger, H., Froestl, W., and Bettler, B. (1997) Nature 386 239-246 [DOI] [PubMed] [Google Scholar]
- 28.Schaeren-Wiemers, N., and Gerfin-Moser, A. (1993) Histochemistry 100 431-440 [DOI] [PubMed] [Google Scholar]
- 29.Pagano, A., Rovelli, G., Mosbacher, J., Lohmann, T., Duthey, B., Stauffer, D., Ristig, D., Schuler, V., Meigel, I., Lampert, C., Stein, T., Prezeau, L., Blahos, J., Pin, J., Froestl, W., Kuhn, R., Heid, J., Kaupmann, K., and Bettler, B. (2001) J. Neurosci. 21 1189-1202 [DOI] [PMC free article] [PubMed] [Google Scholar]
- 30.Rolink, A. G., Andersson, J., and Melchers, F. (1998) Eur. J. Immunol. 28 3738-3748 [DOI] [PubMed] [Google Scholar]
- 31.Goslin, K., Asmussen, H., and Banker, G. (1998) in Culturing Nerve Cells (Banker, G., and Goslin, K., eds) pp. 339-370, MIT Press, Cambridge, MA
- 32.Bischoff, S., Leonhard, S., Reymann, N., Schuler, V., Shigemoto, R., Kaupmann, K., and Bettler, B. (1999) J. Comp. Neurol. 412 1-16 [PubMed] [Google Scholar]
- 33.Lei, S., and McBain, C. J. (2003) J. Physiol. (Lond.) 546 439-453 [DOI] [PMC free article] [PubMed] [Google Scholar]
- 34.Dunlap, K. (1981) Br. J. Pharmacol. 74 579-585 [DOI] [PMC free article] [PubMed] [Google Scholar]
- 35.Saglietti, L., Dequidt, C., Kamieniarz, K., Rousset, M. C., Valnegri, P., Thoumine, O., Beretta, F., Fagni, L., Choquet, D., Sala, C., Sheng, M., and Passafaro, M. (2007) Neuron 54 461-477 [DOI] [PubMed] [Google Scholar]
- 36.Sia, G. M., Beique, J. C., Rumbaugh, G., Cho, R., Worley, P. F., and Huganir, R. L. (2007) Neuron 55 87-102 [DOI] [PubMed] [Google Scholar]
- 37.Gally, C., Eimer, S., Richmond, J. E., and Bessereau, J. L. (2004) Nature 431 578-582 [DOI] [PMC free article] [PubMed] [Google Scholar]
- 38.Fairfax, B. P., Pitcher, J. A., Scott, M. G., Calver, A. R., Pangalos, M. N., Moss, S. J., and Couve, A. (2004) J. Biol. Chem. 279 12565-12573 [DOI] [PubMed] [Google Scholar]
- 39.Froestl, W., Gallagher, M., Jenkins, H., Madrid, A., Melcher, T., Teichman, S., Mondadori, C. G., and Pearlman, R. (2004) Biochem. Pharmacol. 68 1479-1487 [DOI] [PubMed] [Google Scholar]
Associated Data
This section collects any data citations, data availability statements, or supplementary materials included in this article.