Abstract
ATP-binding cassette transporters play an important role in drug resistance and nutrient transport. In the human malaria parasite Plasmodium falciparum, a homolog of the human p-glycoprotein (PfPgh-1) was shown to be involved in resistance to several drugs. More recently, many transporters were associated with higher IC50 levels in responses to chloroquine (CQ) and quinine (QN) in field isolates. Subsequent studies, however, could not confirm the associations, although inaccuracy in drug tests in the later studies could contribute to the lack of associations. Here we disrupted a gene encoding a putative multidrug resistance-associated protein (PfMRP) that was previously shown to be associated with P. falciparum responses to CQ and QN. Parasites with disrupted PfMRP (W2/MRPΔ) could not grow to a parasitemia higher than 5% under normal culture conditions, possibly because of lower efficiency in removing toxic metabolites. The W2/MRPΔ parasite also accumulated more radioactive glutathione, CQ, and QN and became more sensitive to multiple antimalarial drugs, including CQ, QN, artemisinin, piperaquine, and primaquine. PfMRP was localized on the parasite surface membrane, within membrane-bound vesicles, and along the straight side of the D-shaped stage II gametocytes. The results suggest that PfMRP plays a role in the efflux of glutathione, CQ, and QN and contributes to parasite responses to multiple antimalarial drugs, possibly by pumping drugs outside the parasite.
Genes encoding ATP-binding cassette (ABC)2 transporters belong to a supergene family present in organisms from prokaryotes to mammals (1, 2). These genes encode transmembrane proteins that can transport a wide variety of substrates across extra- and intracellular membranes, including metabolic products, lipids, sterols, and drugs; changes in the protein sequences or expressional levels of ABC transporters have been linked to alteration in stress response, cellular detoxification, and various diseases and disorders (3–7). Mutations and/or overexpression of many ABC transporters can also lead to drug resistance in disease-causing microbes and to treatment failure of human cancer and other diseases (8–11).
The genome of the human malaria parasite Plasmodium falciparum contains at least 15 predicted genes encoding putative ABC transporters (12). In particular, mutations and amplification of the gene encoding a homolog of the human P-glycoprotein (PfPgh-1) has been shown to contribute to or associated with parasite responses to chloroquine (CQ), mefloquine (MQ), and quinine (QN) (13–18). Overexpression of another putative ABC transporter (PfMDR2) was also linked to parasite responses to CQ and MQ, but no additional evidence has been obtained to support a role for PfMDR2 in CQ and MQ response since the earliest descriptions (19, 20). Recently, PfMDR2 was found to transport heavy metal and to play a role in heavy metal resistance in P. falciparum (21).
Many drug resistances are complex phenotypes involving multiple genes (16, 22); however, a limited number of genes usually play a key role in determining a drug phenotype. One example is that mutations in the gene encoding a putative P. falciparum chloroquine resistance transporter (PfCRT) can convert a clinical CQ-sensitive (CQS) P. falciparum parasite into a CQ-resistant (CQR) one (23); however, parasites with the same mutant PfCRT allele can display very different levels of resistance to CQ, suggesting contribution from proteins such as PfPgh-1 and other molecules (24, 25). Indeed, mutations in genes encoding several putative transporters (other than PfPgh-1 and PfMDR2) were found to be associated with higher CQ and/or QN half-maximal inhibitory concentration (IC50) among P. falciparum isolates (16, 24). These transporters may play a role in modulating the levels of parasite response to antimalarial drugs.
One of the putative transporters (PFA0590w, also called G2 in Ref. 24) associated with responses to CQ and QN is a member of the ABC transporter C subfamily. ABC transporters in this subfamily are also known as multidrug resistance-associated proteins (MRPs) that can also transport substrates such as glutathione (GSH), glucuronate, and sulfate conjugates as well as various drugs (26). The gene (pfmrp) was reported from P. falciparum previously, and the expression of its mRNA and protein were investigated (27). PfMRP is encoded by a single exon of 5469 bp with 11 predicted transmembrane α-helix segments and is expressed from early trophozoite to late schizont, according to microarray analyses (28–30). Two mutations in the PfMRP were associated with higher levels of IC50 to CQ and QN in P. falciparum field isolates, with good correlation of the mutations in the gene and higher IC50 (24); however, whether PfMRP contributes to parasite drug responses remains controversial (31, 32) and requires additional functional studies.
To further investigate the functions of PfMRP in metabolite transport and drug resistance in malaria parasites and to resolve the discrepancies among different association studies, we have disrupted the putative transporter PfMRP in the P. falciparum parasite. We showed that when the gene encoding the PfMRP in a CQR parasite (W2) was disrupted, the parasite growth was affected and became more sensitive to multiple antimalarial drugs. The pfmrp knock-out parasite also accumulated more CQ and QN compared with its wild type (WT) parasite W2. Our study showed that PfMRP played a role in parasite response to CQ, QN, and other drugs and provided information for better understanding the functions of the PfMRP in transporting antimalarial drugs and other metabolites.
EXPERIMENTAL PROCEDURES
Parasite and Parasite Culture—P. falciparum parasites used in this study have been described (24, 33). The parasites were cultured in vitro according to the methods of Trager and Jensen (34). Briefly, parasites were maintained in RPMI 1640 medium containing 25 mm HEPES, 5% human O+ erythrocytes (5% hematocrit), 0.5% Albumax (Invitrogen), 24 mm sodium bicarbonate, and 10 μg/ml gentamycin at 37 °C with 5% CO2, 5% O2, and 90% N2 with daily medium changes.
Antibody Production and Western Blotting—Polyclonal antibodies against PfMRP were obtained using DNA vaccination. DNA segments encoding two relatively hydrophilic peptides were amplified and cloned separately into VR2001/MRP plasmid DNA (35) for immunization (Fig. 1A). Five-month-old female Swiss Webster mice were injected three times subcutaneously into the tails with 40 μg of purified plasmid DNA (35) at 3-week intervals under National Institutes of Health animal protocol LMVR85E. Blood was collected from the tail vein of the injected animals, and sera were tested for binding to PfMRP on Western blot. Preimmune sera were collected 1 day prior to the first plasmid injection. Antibodies against PfMSP-1, PfCRT, and PfMDV-1 were described previously (23, 33, 36). Parasite proteins were prepared by treatment of parasitized RBCs with 0.1% saponin in PBS on ice for 10 min followed by a cold PBS wash, dissolved in SDS sample loading buffer (50 mm Tris-HCl, pH 6.8, 2% SDS, 10% glycerol, 1% β-mercaptoethanol, 12.5 mm EDTA, 0.02% bromphenol blue) and were separated in 12% SDS-PAGE gels after boiling in sample loading buffer for 5 min. Separated proteins were transferred to a Protran nitrocellulose membrane and probed with antibodies from immunized mice. The signals were developed using horseradish peroxidase-conjugated anti-mouse IgG (1:3000), secondary antibodies diluted in blocking buffer (5% nonfat milk and 0.1% Tween 20 in PBS), and ECL Western blotting detection reagents (Amersham Biosciences) after incubation of the membrane with anti-PfMRP antibodies (1:1000) in blocking buffer at room temperature for 2 h.
FIGURE 1.
Genetic knock-out of PfMRP in W2 P. falciparum parasite. A, Kyte_Doolittle hydrophilicity plot of the predicted amino acid sequence of PfMRP, showing two regions (horizontal bars) used in DNA vaccination to generate antibodies against PfMRP. The x axis is amino acid position, and the y axis is the hydrophilicity score. B, diagram showing a plasmid construct used to disrupt the gene encoding PfMRP. hdhfr is the gene encoding human dihydrofolate reductase, and amp is the gene encoding ampicillin resistance protein. The arrowheads (F1 and R1) indicate PCR primer positions before and after integration of the plasmid into chromosome. Restriction sites and predicted PCR product sizes are as marked. C, PCR products amplified from wild type W2 and W2/MRPΔ parasites using primers F1 and R1 in B. MW, molecular weight markers. The sizes of the PCR products were as expected from DNA sequences with or without plasmid integration, respectively. D, Western blot showing a 214-kDa band in W2 but not in W2/MRPΔ using mouse anti-PfMRP antibodies; no bands were detected in both W2 and W2/MRPΔ using mouse preimmune sera. Anti-PfCRT was used as loading control.
Construction of Transfection Vectors—The plasmid pHD22Y was obtained from Dr. Thomas E. Wellems (37). A segment of 956-bp DNA (132–1068 bp from the 5′ end of the coding region) of the gene was amplified from W2 genomic DNA using PCR primers 5′-GCACTGCAGGGAGATATTCAAGAACT-3′ and 5′-GCAGCGGCCGCGGACAACCATATAGC-3′. The amplified DNA was digested with the restriction enzymes PstI and NotI and cloned into the pHD22Y vector (Fig. 1B). The DNA sequences of all inserts were confirmed by DNA sequencing.
Parasite Transformation and Selection—Asexual stages of W2 parasite were cultured as described above. The parasites were synchronized using 5% d-sorbitol, and schizont stages at 8–10% parasitemia were purified using a Percoll-sorbitol separation method (38, 39). Uninfected RBCs were electroporated with 200 μg of supercoiled pHD22Y containing DNA inserts as described (33, 40). Following transformation, the parasites were maintained in drug-free medium for 48 h before the addition of WR99210 (Jacobus Pharmaceuticals) to a final concentration of 10 nm. Drug-resistant parasites appeared in 3–4 weeks after transfection. DNA samples from the parasite cultures 6–8 weeks after transfection were tested for the presence of integration of plasmid DNA into chromosomes using PCR (forward primer F 5′-GATTGGATAAGACCGTTAATA-3′ and reverse primer R 5′-TAAACTTGGTAAAAATTCAAATAG-3′) (Fig. 1B). When evidence of integration was detected (see Fig. 1C), the parasites were grown without the drug for 2 weeks and then with drug selection for 2 weeks. The procedure was done twice before limiting dilution to clone parasites with disrupted pfmrp.
Parasite Growth and Invasion Rate Assays—A culture of synchronous ring stage was diluted to 0.1% parasitemia and 2% hematocrit. Blood smears were prepared every 24 h over a period of 10 days, and parasitemia were counted under a light microscope after Giemsa staining. Culture media were changed daily or twice a day without the addition of red blood cells until the cultures crashed because of high parasitemia. The invasion rates were calculated by dividing the number of successfully invaded parasites with the initial parasitemia after one cycle (48 h). The experiment was repeated four times.
Immunofluorescence Assay—Glass slides with parasite smear were fixed in cold methanol (in dry ice) for 15 min and dried at room temperature for 15 min. The samples were blocked with blocking buffer (5% nonfat milk in PBS) at room temperature for 2 h. The slides were incubated with mouse primary antibody against PfMRP (diluted 1:200) at room temperature for 2 h or 4 °C overnight and then with diluted goat anti-mouse or anti-rabbit antibodies (1:1000) at room temperature for 30 min after washing the slides three times with blocking buffer. The slides were mounted with ProLong Gold antifade reagent with 4′,6′-diamino-2-phenylindole (Invitrogen) after washing five times with buffer and were observed under a confocal microscope (Leica SP2, Leica Microsystems, Exton, PA) using a 100× oil immersion objective NA 1.4. The images were deconvolved with Huygens Essential software (version 3.1; Scientific Volume Imaging BV, Hilversum, The Netherlands). Sequential Z-sections of stained cells were also collected for three-dimensional reconstruction and iso-surface modeling of representative cells with Imaris software (version 6.0; Bitplane AG, Zurich, Switzerland).
Drug Assays—CQ, QN, MQ, artemisinin (ART), and primaquine (PRQ) were purchased from Sigma-Aldrich; amodiaquine (AMQ) was bought from LGC Promochem; piperaquine (PQ) was obtained as a gift from Dr. Xinhua Wang (Guanzhou University of Traditional Chinese Medicine, China); and proguanil (PG) was obtained from the National Institutes of Health pharmacy. CQ, QN, MQ, ART, PG, and AMQ were dissolved in 70% ethanol and stored at -80 °C until use. PRQ was dissolved in water, stored at 4 °C, and used within a week. PQ was dissolved in 0.5% lactic acid and stored at -80 °C until use. Drug assays were performed using a SYBR green staining method modified from that described previously (41). Briefly, the parasites were diluted to 1% parasitemia with 1% hematocrit, and diluted parasites (150 μl) were added to wells in triplicate in a 96-well plate containing 50 μl of 2-fold serially diluted drugs. The parasites were incubated with the drugs at 37 °C for 72 h. After incubation, DNA were released and stained with lysis buffer containing SYBR green dye. The plate was kept in darkness for 30 min and read in a FLUOstar Optima microplate reader (BMG Labtech). All of the data points from each parasite and dilution were independently repeated at least three times.
Drug Accumulation Assay—Highly synchronized trophozoite parasites (parasitemia of 4–6% and hematocrits of 5.0%) were washed with PBS and then incubated in RPMI 1640 medium supplemented by 25 mm HEPES (pH 7.35), 0.2% NaHCO3, 0.2% d-glucose, 1% human serum, and radioactive-labeled CQ (20 nm [3-3H]), QN (2 nm [9-3H]), and reduced GSH ([glycine-2-3H] at 1, 3, and 6 nm) (American Radiolabeled Chemicals) at 37 °C for 5–60 min under continuous stirring. The parasites were centrifuged through silicon oil (Fulka Chemical Corp.) (density, 1.049) in 1.5-ml Eppendorf tubes, separating the RBC pellet from unincorporated label. The bottom tips of the tubes containing the cell pellets were cut off and placed in scintillation vials containing 100 μl of ethanol and 50 μl of 0.5 n NCS-II tissue solubilizer solution (Amersham Biosciences). The lysates were incubated at 37 °C overnight and were decolorized by the addition of 25 μl of 30% H2O2. Luminescence was blocked by acidification with 25 μl of glacial acetic acid. Radioactive levels were measured in a liquid scintillation counter (1450 MicroBeta TriLux; PerkinElmer Life Sciences). Uninfected RBCs and controls were subjected to identical protocols. Data from different experiments were normalized for differences in hematocrit, parasitemia, and radioactive labels.
Data Analysis and IC50 Calculation—Data from microplate reader were analyzed using GraphPad Prism (La Jolla, CA) or R software (version 2.7.0) (42). The IC50 values were estimated using 4 parameter logistic models, where for the computations the control (i.e. drug concentration = 0) and uninfected RBC (acting like an extremely large drug concentration that stops all growth) were set to 10-20 m and 1010 m, respectively. The model for the percentage of inhibition is b + (100 - b)/[1 + 10(d-X)*H], where X is the log transformed drug concentration, and the parameters b (background inhibition), d (logIC50), and H (Hill coefficient) are estimated by least squares. A separate IC50 was calculated for W2 and W2/MRPΔ for each experiment, and a paired t test on the log(IC50) values was used together with the associated confidence intervals.
RESULTS
Generation of Antibodies against PfMRP—The predicted DNA sequence encoding PfMRP was downloaded from PlasmoDB, and two DNA segments encoding regions of the protein likely to be exposed on membrane surface (hydrophilic) were selected (codons 475–705 and 806–1120) as targets of DNA vaccination (Fig. 1A). The DNA segments were cloned into the VR2001 plasmid vector (35) and were used to immunize mice separately. After three injections of plasmid constructs containing the target sequences, sera from five mice for each construct were collected and tested for antibodies against PfMRP in parasite lysates. All of the mice produced antibodies against PfMRP when tested on Western blots (data not shown). The antibodies from the region of codon 806–1120 gave stronger signals in Western blot and were used in all the experiments. It appeared to be specific for PfMRP because only one major band with predicted molecular weight was detected on the Western blot when tested using pooled antisera against PfMRP (Fig. 1D).
Genetic Knock-out Gene Encoding PfMRP—To investigate the biological functions of PfMRP and whether it plays a role in transporting drugs and in parasite resistance to antimalarial drugs, we disrupted the coding region of PfMRP by inserting a plasmid (pHD22Y) cassette containing a gene encoding human dihydrofolate reductase into the 5′ PfMRP coding region (37). Amplification of DNA segment using primers flanking the targeted sequence showed the presence of parasites with integration of the plasmid sequence in the transfected parasites. Insertion of the plasmid construct produced a PCR product of 8.68 kilobase pairs from the parasite with disrupted pfmrp, whereas the WT parasite had a PCR product of 1020 bp (Fig. 1C). Limiting dilution was performed to identify parasite clones with integration of the plasmid construct into the chromosome that disrupt the coding region of PfMRP. Five weeks after cloning, two clones of parasites with disrupted PfMRP were obtained. The absence of protein expression in the W2/MRPΔ parasites was confirmed using antibodies against PfMRP from DNA vaccination described above (Fig. 1D).
No Changes in pfcrt, pfmdr1, and a Gene Encoding a Putative Sodium Hydrogen Exchanger (PfNHE) in the W2/MRPΔ Parasite—Cross-contamination of parasites during in vitro culture occurs frequently. To confirm the identities of the knock-out parasite clones, we genotyped DNA from W2 and W2/MRPΔ clones using 10 highly polymorphic microsatellite markers (data not shown and supplemental Table 1) (43) and a multicopy molecular fingerprinting marker PfRRM (supplemental Fig. 1) (44). No differences were observed between W2 and two W2/MRPΔ clones, confirming that the W2/MRPΔ clones indeed derived from W2.
Various putative parasite transporters have been associated with drug resistances. In P. falciparum, PfCRT, PfPgh-1, and PfNHE have been associated with responses to CQ, QN, MQ, and other drugs (16, 45). To rule out the possibility that the observed changes in drug responses and impaired growth at high parasitemia in the W2/MRPΔ were caused by changes in these genes, we compared DNA sequences encoding PfCRT, PfPgh-1, and PfNHE from W2 and W2/MRPΔ. No changes in the genes were found (data not shown).
Impaired Parasite Growth in Vitro after Disruption of PfMRP— Although the W2/MRPΔ parasite appeared to grow normally, it could not grow to a density higher than 5% parasitemia if culture medium was changed once a day, whereas the WT parasite W2 could be routinely cultured up to 15% parasitemia under the same conditions (Fig. 2A). The impaired growth capability of the W2/MRPΔ was not due to lower efficiency in invading RBCs. We counted the numbers of newly invaded parasites for the first invasion cycle and found that the ratios of the numbers of ring stage over the initial numbers of parasites were similar for both W2 and W2/MRPΔ (4.85 ± 0.40 S.D. for W2/MRPΔ versus 5.27 ± 0.51 S.D. for W2). The growth impairment could be due to lower efficiency in removing toxic metabolites from inside the parasite cells or due to reduced ability in acquisition of nutrients from the culture medium. Indeed, change of culture medium twice a day (but no addition of red blood cells) increased the maximum parasitemia of the W2/MRPΔ to ∼7% (Fig. 2A). Because the same culture medium has been routinely used to grow various parasite isolates (including W2) to more than 10% parasitemia, it was unlikely that the W2/MRPΔ parasite growth inhibition was due to a lack of necessary nutrients in the medium. Instead, disruption of PfMRP may impair the ability of the parasite to remove toxic metabolites outside the cell, because MRP is known to efflux GSH conjugates and other metabolites (46, 47).
FIGURE 2.
Impaired asexual growth and changes in sexual development in the W2/MRPΔ parasite. A, synchronized wild type W2 and W2/MRPΔ parasites were diluted to 0.1% parasitemia and allowed to grow with change of media once or twice a day (without the addition of fresh red blood cells). W2/MRPΔ-1 and W2–1 were grown in culture with medium change once a day. W2/MRPΔ-2 and W2–2 were the same parasites, but grown in cultures with medium changes twice a day. Parasite cultures “crashed” after day 8 or 9, particularly for those of wild type parasites. B, gametocytemia from W2 and W2/MRPΔ parasites; stage II–V gametocytes were counted daily. The data points in the figures were averages from three independent experiments, and the bars indicate standard deviations.
Disruption of PfMRP and the reduced ability to transport toxic metabolites may make the parasite more sensitive to environmental stresses, which could in turn affect parasite sexual development, including the switch from asexual stages into gametocyte (48). Similar numbers of mature gametocytes (∼2% parasitemia at day 10) were obtained from both W2 and W2/MRPΔ parasites (Fig. 2B); however, young gametocytes (stage II) were seen 2–3 days earlier in W2/MRPΔ than in W2 after setting up gametocyte cultures starting at 0.1% parasitemia. In contrast to a gradual accumulation of stage II or later gametocyte stages (recognizable) in W2/MRPΔ from days 4 to 8 after setting up gametocyte culture, there was a rapid increase (suggesting switching to sexual development approximately at the same time) in the number of gametocytes in W2 at day 7–8 (Fig. 2B). These results indicated that W2/MRPΔ was more sensitive to environmental stresses and that some parasites were triggered to switch at conditions that were still “normal” for WT W2.
Increased GSH Accumulation in the W2/MRPΔ Parasite— MRP has been shown to transport GSH and its conjugates such as GSSG, GSH drug adducts, and other metabolites outside mammalian cells (47). The ability to transport GSH conjugates outside the parasite cell may be related to how parasites respond to stresses caused by accumulation of metabolic toxins in in vitro culture, which may explain why the W2/MRPΔ could not grow to a parasitemia higher than 5% when culture medium was changed once a day. We therefore investigated GSH accumulation in 3D7, W2/MRPΔ, and W2 parasites. 3D7 is a CQS parasite that has wild type PfCRT and PfMRP and was included as a control for PfCRT effects on drug accumulation. For all the parasites, accumulation of GSH peaked at ∼20 min after addition of radioactive GSH, and the GSH concentrations stayed at similar levels until the experiments were terminated at 60 min (Fig. 3). Although W2 and 3D7 accumulated approximately the same amount of radioactive GSH, the W2/MRPΔ parasite accumulated approximately twice as much radiolabeled GSH as the WT W2 did (Fig. 3), suggesting that PfMRP may play a role in pumping GSH or GSH conjugates outside the cell and that disruption of PfMRP affects the transport process. RBC accumulated very minimum radioactive GSH, possibly because of active transport of GSH by human MRP and other transporters present on the RBC membrane.
FIGURE 3.
GSH accumulation assays. Parasitized RBCs or uninfected RBCs were incubated with radioactive GSH (the curves shown are from 3 nm GSH), and the radioactivities in the RBCs were measured at different time points. Unincorporated radioactive materials were removed by spinning the RBC through silicon oil. The data were from three independent repeats. Similar accumulation curve patterns were obtained using 1 and 6 nm radioactive labeled GSH (data not shown).
Increased CQ and QN Accumulation in the W2/MRPΔ Parasite—One of the mechanisms for many ABC transporters to confer drug resistances is to rapidly pump drugs outside the cells (4, 10, 11). To investigate the possibility of PfMRP playing a role in drug efflux, we performed drug accumulation assays comparing W2, W2/MRPΔ, and 3D7. The parasites were incubated with radioactively labeled CQ and QN, and the radioactivity within the parasites was measured at various time points. Compared with the W2 parasite, the W2/MRPΔ parasite accumulated more radioactive labeled CQ (59.0%) and QN (55.2%) than W2 did (Fig. 4), suggesting that PfMRP plays a role in pumping CQ and QN outside the parasite cell. The CQS 3D7 parasite accumulated approximately two to three times more CQ or QN than both W2 and W2/MRPΔ did, whereas RBC accumulated minimum radioactive CQ and QN. Accumulation of more radioactive CQ and QN in 3D7 could be due to retention of more CQ and QN in the parasite food vacuole, because 3D7 has a WT PfCRT, making the drug less accessible to PfMRP and other transporters.
FIGURE 4.
CQ and QN accumulation assays with or without GSH. A, CQ accumulation. B, CQ radioactivity at 30 min. C, QN accumulation. D, QN radioactivity at 30 min. Parasites and treatments with or without GSH are as indicated. The standard deviations were from three independent experiments.
CQ and QN Accumulations Differentially Affected by GSH—Because disruption of PfMRP appeared to affect the transport of both GSH and CQ/QN, it would be interesting to investigate how gene disruption affects drug transport in the presence of exogenous GSH because increased GSH levels in P. falciparum-infected cells were reported to increase parasite resistance to CQ (49). Of interest, the presence of 2 mm exogenous GSH dramatically reduced CQ accumulation in the CQS parasite 3D7 (47.8% reduction) at 30 min but had little effect on CQ accumulation of CQR parasite W2 (11.4%) (Fig. 4, A and B). The level of CQ accumulation was also reduced 30.3% in the W2/MRPΔ parasite at the presence of GSH. In contrast, the presence of GSH dramatically reduced QN accumulation in W2 (83.6%) as well as in 3D7 (65.1%) (Fig. 4, C and D). Similarly, partial reduction (37.4%) of QN accumulation was observed in the W2/MRPΔ parasite (Fig. 4, C and D). These results indicated that GSH could enhance the transport of drugs outside the parasite cell at least in some parasites, possibly through pumping of GSH drug adducts by PfMRP and other transporters. However, because the reduction in CQ accumulation was mostly found in the W2/MRPΔ parasite, the GSH effect was more likely on transporters other than PfMRP.
Increased Susceptibility to Multiple Antimalarial Drugs after PfMRP Disruption—Increased CQ and QN accumulation after disruption of PfMRP suggested that it played a role in pumping the drugs outside the parasite, which may lead to reduced susceptibility to the drugs. To investigate whether PfMRP plays a role in parasite susceptibility to antimalarial drugs, we tested the IC50 of W2 and W2/MRPΔ parasites in responses to eight different drugs, including CQ, QN, MQ, ART, PQ, AMQ, PRQ, and PG using a SYBR in vitro test. Compared with its WT W2, the W2/MRPΔ parasite became significantly more sensitive to five of the eight drugs tested (CQ, QN, ART, PQ, and PRQ; Table 1). These results showed that PfMRP played a role in parasite response to multiple antimalarial drugs, possibly by transporting the drugs outside the parasite cell; however, PfMRP might not be a key molecule that can convert a clinically sensitive parasite into a resistant parasite, because the five significant changes in IC50 were only 38–57% reductions.
TABLE 1.
IC50 of W2 and W2/MRPΔ parasites in responses to eight antimalarial drugs W2Δ is the abbreviated name for W2/MRPΔ, The IC50 values are geometric means (nm), and % Change is 100(W2Δ IC50 – W2 IC50)/W2 IC50. LCI and UCI are lower and upper confidence intervals, respectively. The p values and CIs on the % change are derived from the paired t test on the log(IC50) values. Note that CQ, QN, ART, PQ, and PRQ are significant even after correcting for multiple tests using Bonferroni's adjustment (p < 0.05).
Drug | W2/IC50 | W2Δ/IC50 | % Change | 95% LCI | 95% UCI | No. repeats | Two-sided p value |
---|---|---|---|---|---|---|---|
CQ | 163 | 101 | –38 | –45 | –31 | 8 | <0.01 |
QN | 174 | 76 | –56 | –62 | –49 | 8 | <0.01 |
AMQ | 67 | 60 | –10 | –21 | 3 | 4 | 0.01 |
MQ | 12 | 11 | –7 | –22 | 11 | 8 | 0.37 |
ART | 11 | 5 | –57 | –61 | –52 | 9 | <0.01 |
PQ | 138 | 82 | –40 | –50 | –29 | 4 | <0.01 |
PG | 104 | 64 | –38 | –75 | 53 | 4 | 0.19 |
PRQ | 2660 | 1243 | –53 | –63 | –42 | 4 | <0.01 |
Presence of Exogenous GSH, but Not GSSG, Increases IC50 to CQ and QN in Both W2 and W2/MRPΔ Parasites—The presence of exogenous GSH increased the IC50 to both CQ and QN partly because of reduced accumulation of the drugs (Fig. 5, A and B). The presence of GSSG, however, had no or minimum effects on both CQ and QN IC50 (Fig. 5, C and D). The increase in IC50 in W2/MRPΔ parasite suggested that genes other than PfMRP were also involved in the drug response at the presence of GSH. These may include other unidentified transporters or the degradation of heme by GSH (49).
FIGURE 5.
Changes in responses to CQ and QN at the presence of exogenous GSH (2.5 mm) and GSSG (5.0 mm). A and C, response to CQ. B and D, response to QN. The parasite groups used are as indicated in the figures. The data presented were averages from three independent repeats. The graphs were plotted using a nonlinear regression model in GraphPad Prism 5 (La Jolla, CA) with constraint at the top of the graphs but no constraint at the bottom.
Expression and Localization of PfMRP in Asexual and Sexual Stages—PfMRP was predicted to be a membrane protein with 11 transmembrane domains and was transcribed in both asexual and sexual stages (28–30). A previous study also suggested that PfMRP was expressed on the plasma membrane of trophozoite and schizont stages but was not detectable at ring stage or on the membrane of food vacuole (27). To further characterize the protein expression patterns and localization of PfMRP in different developmental stages, we performed immunofluorescent assay using antibodies against PfMRP, PfMSP-1 (a protein on merozoite plasma membrane), PfCRT (on food vacuole membrane), and PfMDV-1 (gametocyte-specific). We found that PfMRP was expressed in all of the asexual and sexual stages that we investigated, from merozoite to mature schizont, and from young gametocyte to mature gametocyte (Figs. 6 and 7; supplemental Fig. 2).
FIGURE 6.
Expression and localization of PfMRP within asexual stages. Row 1, green, anti-PfMRP; row 2, red, anti-PfMSP-1; row 3, blue, 4′,6′-diamino-2-phenylindole dye; row 4, merged rows 1–3; row 5, merged rows 1–4 and differential interference contrast. A, free merozoites of the W2 parasite. B, a trophozoite of the W2 parasite. C, a schizont of the W2 parasite. D, three trophozoites of the W2/MRPΔ parasites within a red blood cell. E, a schizont of the W2/MRPΔ parasite.
FIGURE 7.
Localization of PfMRP in sexual stages. Row 1, green, anti-PfMRP; row 2, red, anti-PfMDV-1; row 3, blue, 4′,6′-diamino-2-phenylindole dye; row 4, differential interference contrast; row 5, merged rows 1–4. A, stage I gametocyte identified by anti-PfMDV-1 staining. B, stage II gametocyte showing two broad straight lines stained strongly with anti-PfMRP. C, stage III–V gametocyte showing surface membrane staining and some internal membrane networks. D and E, iso-surface model of the stage II gametocyte reconstructed from serial Z-sections of confocal images. D, side view of a stage II gametocyte showing two heavily stained structures (green) parallel to the straight side of the D-shaped parasite. Internal vesicles with PfMRP (white arrowhead) could also be seen inside the parasite. E, bottom view of the same stage II gametocyte. The red shown in this transparent iso-surface model indicates the distribution of PfMDV-1 that is a gametocyte-specific protein located on gametocyte outside membrane and internal vesicles (33).
In merozoites, PfMRP was expressed in membrane-bound vesicles located inside the parasite plasma membrane and did not co-localize with PfMSP-1 on plasma membrane (Fig. 6A) or PfCRT on food vacuole membrane (supplemental Fig. 2). In trophozoite and schizont stages, PfMRP expression increased with parasite growth, located on an extensive network of membrane structures including plasma membrane, although it appeared to be present in “patches” (Fig. 6, B and C). PfMRP was not detected in W2/MRPΔ when probed with anti-PfMRP antibodies (Fig. 6, D and E). The residual staining seen in Fig. 6E appeared to be nonspecific binding to hemozoin.
PfMRP was also highly expressed in various sexual stages. In young gametocytes (identified by anti-PfMDV-1), PfMRP was mostly expressed on the plasma membrane of the parasite (Fig. 7A), although some staining could also be seen in membrane-bound vesicles or networks inside the cell (data not shown). As the parasite developed into D-shaped stage II, the protein was concentrated at the “straight” side of the D-shaped parasite, showing as one or two heavily stained “lines” (Fig. 7, B and D). Three-dimensional reconstruction of thin confocal sections from stage II gametocyte showed that one of the double straight lines was likely located at the parasite plasma membrane that might be associated with the inner pellicular membrane vacuole and microtubule structures that contributes to the D-shaped morphology (50). The second line was parallel to the outside line, which could represent an unknown membrane structure in stage II gametocytes (Fig. 7, D and E). Similarly to those in asexual stages, the protein is also present in membrane-bound vesicles or networks in the parasite (Fig. 7D). In later gametocyte stages (III–VI), PfMRP continued to be present on plasma membrane and in some internal membrane networks (Fig. 7C).
DISCUSSION
Two point mutations at amino acid positions 191 (His → Tyr) and 437 (Ser → Ala) in PfMRP were associated with higher IC50 for CQ and QN in P. falciparum field isolates previously (24). In particular, parasites carrying mutant alleles Tyr191 and Ala437 appeared to be more resistant to QN in parasites from the South America (supplemental Table S2). Except JAV and ECU, the parasites from Brazil and Peru had very similar genetic backgrounds and minimum population structure based on analyses using hundreds of microsatellites and single nucleotide polymorphism on chromosome 3 (51, 52). Although the parasite sample size was small in the study, a pattern can be seen in QN responses for parasites from South America when parasite responses to the drug were repeatedly tested in vitro. Two subsequent studies of in vitro drug assays on parasites collected directly from patients, however, did not support the associations (31, 32). To resolve the differences, more association studies using parasites from the same location and accurate drug assays on culture-adapted parasites are necessary. Another approach to resolve the controversy is to change the level of gene expression or to totally knock out the gene from the parasite genome and investigate the resulting effects. Here we successfully knocked out the gene encoding PfMRP from a CQR parasite W2, resulting in a parasite (W2/MRPΔ) that was more sensitive to CQ and QN than was W2. The data suggested that PfMRP played a role in parasite responses to CQ and QN, supporting the associations of mutations in PfMRP and increased IC50 to CQ and QN, although it may not be a key molecule in resistance to CQ and QN.
The changes in IC50 of various antimalarial drugs in the W2/MRPΔ parasite were likely due to altered ability of the parasite to pump out the drugs, because disruption of PfMRP resulted in accumulation of more CQ and QN. Our data and others (27) also showed that, in addition to being in vesicles of merozoite, ring, and trophozoite stages, the PfMRP protein appeared to be present on the plasma membrane of various parasite stages. Mutations in the CQ transporter gene (pfcrt) have been shown to result in accumulation of less CQ in the parasite food vacuole (53). Because the PfCRT is localized on the membrane of the food vacuole, efflux of CQ out of the parasite plasma membrane may require additional transport mechanism(s) to move CQ out of the cell. It is possible for PfMRP to act as a molecular pump, pumping drugs outside the parasite cell. Indeed, our CQ and QN accumulation assay showed that parasites with disrupted PfMRP accumulated more CQ and QN than WT W2 did, supporting the hypothesis that PfMRP is a molecular pump capable of pumping drugs and other metabolites outside the parasite. The small changes (∼2-fold) in the IC50 values, however, suggested that PfMRP was not the only protein or mechanism providing this function.
In addition to changes in IC50 of CQ and QN, disruption of the gene encoding PfMRP also led to significant changes in parasite sensitivities to three other antimalarial drugs that likely have different modes of action (Table 1). Of particular interest is the significant reduction in ART IC50 in the W2/MRPΔ parasite. The decline in the efficacy of ART-based treatment among field isolates has received great attention recently (54). Changes in parasite responses to these drugs suggested that PfMRP was capable of transporting multiple drugs. The parasite with disrupted PfMRP had an impaired growth capability and was not able to grow to a density higher than 5% parasitemia under standard in vitro culture conditions that can routinely support the WT parasite W2 to a density up to 15% parasitemia. This reduced fitness is likely due to the inability of the W2/MRPΔ parasite to efficiently remove toxic metabolites such as reactive oxygen species (ROS). As the density of parasite increases in culture, more ROS such as H2O2 produced either from hemoglobin digestion in the food vacuole, from electronic transport in mitochondrion, or from other metabolic processes will be accumulated inside the parasite and in the culture medium (55, 56). H2O2 can be reduced to H2O and O2 through various processes, including reactions that lead to the production of GSSG and other GSH conjugates. These GSH conjugates are then transported outside the cell by MRP or other transporters (46, 47, 55). Our data showing accumulation of more radioactive GSH, GSSG, and GSH conjugates in the W2/MRPΔ parasite appear to support the hypothesis that disruption of PfMRP impairs the ability of the parasite to pump out GSH conjugates, which leads to weakened capability in reducing ROS inside the parasite cell and to early death of the parasite caused by the rising ROS concentration within the cell and in culture medium.
GSH can also enhance the transport of various drugs, possibly through pumping out GSH drug adducts (57). One example of enhanced drug transport by GSH is the rapid efflux of GSH-4-Nitroquinoline conjugate by MRP (58). Our results of reduced CQ and QN accumulation at the presence of exogenous GSH suggested that a similar mechanism in the efflux of antimalarial drug-GSH conjugates may also play a role. If GSH and CQ/QN are transported through the same transporters, such as PfMRP, GSH is expected to compete with the drugs for the transporting molecules, leading to accumulation of more radioactive CQ/QN inside the cell. In contrast, enhanced transport of CQ/QN in the presence of exogenous GSH supports the idea of GSH drug adduct formation and transport. Furthermore, the presence of GSH might also promote transport (or exchange) of CQ outside of the parasite food vacuole (FV) in a CQS parasite (3D7), through transporters such as PfPgh-1 or even PfCRT that are expressed on the FV membrane. For CQR parasite W2, the transport of CQ out of FV by mutant PfCRT is more efficient than by WT PfCRT, and the effect of GSH on CQ transport out of FV in W2 was minimum (Fig. 4). On the other hand, GSH may greatly enhance the WT PfCRT transport of CQ and QN out of the FV membrane. Mutations in PfCRT have been associated with parasite responses to QN (16, 24) and appear to play a role in QN resistance too. Transport of QN out of FV and/or plasma membrane could rely more on ABC transporters other than PfCRT, resulting in more dramatic reduction in the accumulation of QN in W2 in the presence of GSH. These results suggested different mechanisms in CQ and QN transport and resistance, at least in W2.
GSH was found to degrade free ferriprotoporphorin IX, which is toxic to the parasite. CQ could inhibit the degradation process (49), and parasites resistant to CQ generally had higher levels of GSH (55). Our data also suggested the existence of mechanisms other than those mediated through PfCRT and PfMRP. At the presence of GSH, both W2 and W2/MRPΔ had increased IC50 to CQ and QN, although the W2/MRPΔ parasite had lower IC50 values than W2 had. Because PfMRP was absent in W2/MRPΔ, this increase in IC50 at the presence of GSH could not be explained by the PfMRP mediated transport of the drugs alone. Therefore, increase in IC50 at the presence of GSH might be due to the increased transport of GSH drug adducts outside the cell and the degradation of heme by GSH as suggested (49).
Similar to PfMRP knock-out, allelic exchanges of polymorphic sites in PfPgh-1 (ABC B family) could also affect its substrate specificity, transport, responses to various antimalarial drugs, including CQ, QN, ART, MQ, and other drugs (14, 18, 59). One difference between these two ABC transporters in antimalarial responses is that the mutations in PfPgh-1 usually affect responses to CQ and ART/MQ in opposite directions, whereas disruption of PfMRP makes the parasite more sensitive to CQ, QN, ART, and MQ (not significant; Table 1). Additionally, PfPgh-1 was localized to the food vacuole membrane (60). Potential molecular interactions involving PfMRP and other molecules based on published and our data are summarized in Fig. 8.
FIGURE 8.
Proposed roles of GSH and PfMRP in CQ and other drug resistances in P. falciparum. 1, PfCRT (chloroquine-resistant transporter) plays a key role in CQ resistance, possibly by transporting CQ out of FV. 2, PfPgh-1 may interact with PfCRT and modulate the physiology of FV, which may affect CQ transport. 3, CQ (and quinine) is pumped out of parasite through PfMRP and other molecules, possibly as drug-GSH conjugates. 4, GSH can help remove oxygen radicals such as H2O2. GSSG could be transported out by PfMRP and other transporters. 5, GSH was reported to degrade heme (Fp), a side product from hemoglobin (Hb) digestion. 6, CQ was proposed to inhibit polymerization of Fp into hemozoin (Hz).
Of interest, our study also showed the expression of PfCRT as small “dots” (probably young FVs) in rupturing merozoites, and PfMRP were similarly in membrane-bound vesicles; however, they do not overlap (supplemental Fig. 2). These vesicles could represent proteins in the process of being synthesized and/or transported to plasma membrane. Another interesting observation was the membrane structures stained by anti-PfMRP antibodies along the straight side of the D-shaped stage II gametocytes. P. falciparum gametocytes are classified into five stages (stages I–V), with stage II having a D-shaped morphology. The straight side of the D-shaped morphology is supported by 8–15 microtubules and an inner pellicular membrane vacuole (50). Interestingly, confocal images from immunofluorescent assay showed strong PfMRP staining along the straight side of the D-shaped gametocyte, suggesting potential co-localization of PfMRP with the inner pellicular membrane vacuole. The functional roles for the PfMRP protein expression pattern and the special localization of PfMRP in gametocyte development remain to be determined.
Genetic manipulation of malaria parasites such as genetic knocking out a gene requires relatively long term in vitro culture and drug selection. Background/random mutations could be introduced during this process. Currently, it is impossible to guarantee that no changes other than the disruption of PfMRP occurred in the W2/MRPΔ parasite genome during plasmid introduction and drug selection. The identities of the parasites, however, were verified using microsatellites. We also sequenced genes (pfcrt, pfmdr1, and pfnhe) known to affect or be associated with parasite responses to CQ and QN (16, 18, 23, 61) to ensure that the changes in IC50 and drug accumulation in the W2/MRPΔ parasite were not due to mutations in these genes. Additionally, the phenotypic changes in the W2/MRPΔ are consistent with loss of drug/metabolite transport functions from a molecule such as PfMRP.
In summary, disruption of PfMRP led to a parasite that had reduced fitness in in vitro culture and was more sensitive to multiple antimalarial drugs than the WT was. The changes in these phenotypes were likely due to impaired functions in transporting drugs and toxic metabolites outside the parasite cell after disruption of PfMRP.
Supplementary Material
Acknowledgments
We thank Dr. Juliana Sa for assistance in drug accumulation assay, Dr. Janni Papakrivos for antibodies against PfCRT, Dr. Jesus Valenzuela for assistance in antibody production, Dr. Hagai Ginsburg for helpful discussion, and NIAID intramural editor Brenda Rae Marshall for assistance.
This work was supported, in whole or in part, by the National Institutes of Health Intramural Research Program of the Division of Intramural Research. The costs of publication of this article were defrayed in part by the payment of page charges. This article must therefore be hereby marked “advertisement” in accordance with 18 U.S.C. Section 1734 solely to indicate this fact.
The on-line version of this article (available at http://www.jbc.org) contains supplemental Tables 1 and 2 and supplemental Figs. 1 and 2.
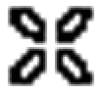
Author's Choice—Final version full access.
Footnotes
The abbreviations used are: ABC, ATP-binding cassette; CQ, chloroquine; QN, quinine; ART, artemisinin; PQ, piperaquine; PRQ, primaquine; MQ, mefloquine; CQS, CQ-sensitive; CQR, CQ-resistant; MRP, multidrug resistance-associated protein; WT, wild type; RBC, red blood cell; PBS, phosphate-buffered saline; AMQ, amodiaquine; PG, proguanil; ROS, reactive oxygen species; FV, food vacuole.
References
- 1.Dean, M., and Annilo, T. (2005) Annu. Re.v Genomics Hum. Genet. 6 123-142 [DOI] [PubMed] [Google Scholar]
- 2.Igarashi, Y., Aoki, K. F., Mamitsuka, H., Kuma, K., and Kanehisa, M. (2004) Mol. Biol. Evol. 21 2149-2160 [DOI] [PubMed] [Google Scholar]
- 3.Stefkova, J., Poledne, R., and Hubacek, J. A. (2004) Physiol. Res. 53 235-243 [PubMed] [Google Scholar]
- 4.Dean, M. (2005) Methods Enzymol. 400 409-429 [DOI] [PubMed] [Google Scholar]
- 5.Ernst, R., Klemm, R., Schmitt, L., and Kuchler, K. (2005) Methods Enzymol. 400 460-484 [DOI] [PubMed] [Google Scholar]
- 6.Yazaki, K. (2006) FEBS Lett. 580 1183-1191 [DOI] [PubMed] [Google Scholar]
- 7.Jungwirth, H., and Kuchler, K. (2006) FEBS Lett. 580 1131-1138 [DOI] [PubMed] [Google Scholar]
- 8.Ouellette, M., Legare, D., and Papadopoulou, B. (1994) Trends Microbiol. 2 407-411 [DOI] [PubMed] [Google Scholar]
- 9.Chakraborti, P. K., Bhatt, K., Banerjee, S. K., and Misra, P. (1999) Biosci. Rep. 19 293-300 [DOI] [PubMed] [Google Scholar]
- 10.Allen, J. D., Brinkhuis, R. F., van Deemter, L., Wijnholds, J., and Schinkel, A. H. (2000) Cancer Res. 60 5761-5766 [PubMed] [Google Scholar]
- 11.Cervenak, J., Andrikovics, H., Ozvegy-Laczka, C., Tordai, A., Nemet, K., Varadi, A., and Sarkadi, B. (2006) Cancer Lett. 234 62-72 [DOI] [PubMed] [Google Scholar]
- 12.Gardner, M. J., Hall, N., Fung, E., White, O., Berriman, M., Hyman, R. W., Carlton, J. M., Pain, A., Nelson, K. E., Bowman, S., Paulsen, I. T., James, K., Eisen, J. A., Rutherford, K., Salzberg, S. L., Craig, A., Kyes, S., Chan, M. S., Nene, V., Shallom, S. J., Suh, B., Peterson, J., Angiuoli, S., Pertea, M., Allen, J., Selengut, J., Haft, D., Mather, M. W., Vaidya, A. B., Martin, D. M., Fairlamb, A. H., Fraunholz, M. J., Roos, D. S., Ralph, S. A., McFadden, G. I., Cummings, L. M., Subramanian, G. M., Mungall, C., Venter, J. C., Carucci, D. J., Hoffman, S. L., Newbold, C., Davis, R. W., Fraser, C. M., and Barrell, B. (2002) Nature 419 498-511 [DOI] [PMC free article] [PubMed] [Google Scholar]
- 13.Foote, S. J., Thompson, J. K., Cowman, A. F., and Kemp, D. J. (1989) Cell 57 921-930 [DOI] [PubMed] [Google Scholar]
- 14.Reed, M. B., Saliba, K. J., Caruana, S. R., Kirk, K., and Cowman, A. F. (2000) Nature 403 906-909 [DOI] [PubMed] [Google Scholar]
- 15.Duraisingh, M. T., Roper, C., Walliker, D., and Warhurst, D. C. (2000) Mol. Microbiol. 36 955-961 [DOI] [PubMed] [Google Scholar]
- 16.Ferdig, M. T., Cooper, R. A., Mu, J., Deng, B., Joy, D. A., Su, X.-Z., and Wellems, T. E. (2004) Mol. Microbiol. 52 985-997 [DOI] [PubMed] [Google Scholar]
- 17.Price, R. N., Uhlemann, A. C., Brockman, A., McGready, R., Ashley, E., Phaipun, L., Patel, R., Laing, K., Looareesuwan, S., White, N. J., Nosten, F., and Krishna, S. (2004) Lancet 364 438-447 [DOI] [PMC free article] [PubMed] [Google Scholar]
- 18.Sidhu, A. B., Valderramos, S. G., and Fidock, D. A. (2005) Mol. Microbiol. 57 913-926 [DOI] [PubMed] [Google Scholar]
- 19.Wilson, C. M., Serrano, A. E., Wasley, A., Bogenschutz, M. P., Shankar, A. H., and Wirth, D. F. (1989) Science 244 1184-1186 [DOI] [PubMed] [Google Scholar]
- 20.Rubio, J. P., and Cowman, A. F. (1994) Exp. Parasitol. 79 137-147 [DOI] [PubMed] [Google Scholar]
- 21.Rosenberg, E., Litus, I., Schwarzfuchs, N., Sinay, R., Schlesinger, P., Golenser, J., Baumeister, S., Lingelbach, K., and Pollack, Y. (2006) J. Biol. Chem. 281 27039-27045 [DOI] [PubMed] [Google Scholar]
- 22.Dent, J. A., Smith, M. M., Vassilatis, D. K., and Avery, L. (2000) Proc. Natl. Acad. Sci. U. S. A. 97 2674-2679 [DOI] [PMC free article] [PubMed] [Google Scholar]
- 23.Fidock, D. A., Nomura, T., Talley, A. K., Cooper, R. A., Dzekunov, S. M., Ferdig, M. T., Ursos, L. M., bir Singh Sidhu, A., Naude, B., Deitsch, K. W., Su, X., Wootton, J. C., Roepe, P. D., and Wellems, T. E. (2000) Mol. Cell 6 861-871 [DOI] [PMC free article] [PubMed] [Google Scholar]
- 24.Mu, J., Ferdig, M. T., Feng, X., Joy, D. A., Duan, J., Furuya, T., Subramanian, G., Aravind, L., Cooper, R. A., Wootton, J. C., Xiong, M., and Su, X.-Z. (2003) Mol. Microbiol. 49 977-989 [DOI] [PubMed] [Google Scholar]
- 25.Duraisingh, M. T., von Seidlein, L. V., Jepson, A., Jones, P., Sambou, I., Pinder, M., and Warhurst, D. C. (2000) Parasitology 121 1-7 [DOI] [PubMed] [Google Scholar]
- 26.Rebbeor, J. F., Connolly, G. C., and Ballatori, N. (2002) Biochim. Biophys. Acta 1559 171-178 [DOI] [PubMed] [Google Scholar]
- 27.Klokouzas, A., Tiffert, T., van Schalkwyk, D., Wu, C. P., van Veen, H. W., Barrand, M. A., and Hladky, S. B. (2004) Biochem. Biophys. Res. Commun. 321 197-201 [DOI] [PubMed] [Google Scholar]
- 28.Le Roch, K. G., Zhou, Y., Blair, P. L., Grainger, M., Moch, J. K., Haynes, J. D., De La Vega, P., Holder, A. A., Batalov, S., Carucci, D. J., and Winzeler, E. A. (2003) Science 301 1503-1508 [DOI] [PubMed] [Google Scholar]
- 29.Bozdech, Z., Llinas, M., Pulliam, B. L., Wong, E. D., Zhu, J., and DeRisi, J. L. (2003) PLoS Biol. 1 E5. [DOI] [PMC free article] [PubMed] [Google Scholar]
- 30.Young, J. A., Fivelman, Q. L., Blair, P. L., de la Vega, P., Le Roch, K. G., Zhou, Y., Carucci, D. J., Baker, D. A., and Winzeler, E. A. (2005) Mol. Biochem. Parasitol. 143 67-79 [DOI] [PubMed] [Google Scholar]
- 31.Anderson, T. J., Nair, S., Qin, H., Singlam, S., Brockman, A., Paiphun, L., and Nosten, F. (2005) Antimicrob. Agents Chemother. 49 2180-2188 [DOI] [PMC free article] [PubMed] [Google Scholar]
- 32.Cojean, S., Noel, A., Garnier, D., Hubert, V., Le Bras, J., and Durand, R. (2006) Malaria J. 5 24. [DOI] [PMC free article] [PubMed] [Google Scholar]
- 33.Furuya, T., Mu, J., Hayton, K., Liu, A., Duan, J., Nkrumah, L., Joy, D. A., Fidock, D. A., Fujioka, H., Vaidya, A. B., Wellems, T. E., and Su, X.-Z. (2005) Proc. Natl. Acad. Sci. U. S. A. 102 16813-16818 [DOI] [PMC free article] [PubMed] [Google Scholar]
- 34.Trager, W., and Jensen, J. B. (1976) Science 193 673-675 [DOI] [PubMed] [Google Scholar]
- 35.Oliveira, F., Kamhawi, S., Seitz, A. E., Pham, V. M., Guigal, P. M., Fischer, L., Ward, J., and Valenzuela, J. G. (2006) Vaccine 24 374-390 [DOI] [PubMed] [Google Scholar]
- 36.Singh, S., Plassmeyer, M., Gaur, D., and Miller, L. H. (2007) Proc. Natl. Acad. Sci. U. S. A. 104 20043-20048 [DOI] [PMC free article] [PubMed] [Google Scholar]
- 37.Fidock, D. A., and Wellems, T. E. (1997) Proc. Natl. Acad. Sci. U. S. A. 94 10931-10936 [DOI] [PMC free article] [PubMed] [Google Scholar]
- 38.Lambros, C., and Vanderberg, J. P. (1979) J. Parasitol. 65 418-420 [PubMed] [Google Scholar]
- 39.Fernandez, V., Treutiger, C. J., Nash, G. B., and Wahlgren, M. (1998) Infect. Immun. 66 2969-2975 [DOI] [PMC free article] [PubMed] [Google Scholar]
- 40.Wu, Y., and Wellems, T. E. (1996) Proc. Natl. Acad. Sci. U. S. A. 93 1130-1134 [DOI] [PMC free article] [PubMed] [Google Scholar]
- 41.Smilkstein, M., Sriwilaijaroen, N., Kelly, J. X., Wilairat, P., and Riscoe, M. (2004) Antimicrob. Agents Chemother. 48 1803-1806 [DOI] [PMC free article] [PubMed] [Google Scholar]
- 42.R Development Core Team (2008) R: A Language and Environment for Statistical Computing, R Foundation for Statistical Computing, Vienna, Austria
- 43.Su, X.-Z., Ferdig, M. T., Huang, Y., Huynh, C. Q., Liu, A., You, J., Wootton, J. C., and Wellems, T. E. (1999) Science 286 1351-1353 [DOI] [PubMed] [Google Scholar]
- 44.Su, X.-Z., Carucci, D. J., and Wellems, T. E. (1998) Exp. Parasitol. 89 262-265 [DOI] [PubMed] [Google Scholar]
- 45.Su, X.-Z., Hayton, K., and Wellems, T. E. (2007) Nat. Rev. Genet. 8 497-506 [DOI] [PubMed] [Google Scholar]
- 46.Rychlik, B., Pulaski, L., Sokal, A., Soszynski, M., and Bartosz, G. (2000) Acta Biochim. Pol. 47 763-772 [PubMed] [Google Scholar]
- 47.Liang, X. J., and Aszalos, A. (2006) Curr. Drug Targets 7 911-921 [DOI] [PubMed] [Google Scholar]
- 48.Dyer, M., and Day, K. P. (2000) Parasitol. Today 16 102-107 [DOI] [PubMed] [Google Scholar]
- 49.Ginsburg, H., Famin, O., Zhang, J., and Krugliak, M. (1998) Biochem. Pharmacol. 56 1305-1313 [DOI] [PubMed] [Google Scholar]
- 50.Sinden, R. E. (1998) in Malaria: Parasite Biology, Pathogenesis, and Protection (Sherman, I. W., ed) American Society for Microbiology, Washington, DC
- 51.Wootton, J. C., Feng, X., Ferdig, M. T., Cooper, R. A., Mu, J., Baruch, D. I., Magill, A. J., and Su, X.-Z. (2002) Nature 418 320-323 [DOI] [PubMed] [Google Scholar]
- 52.Mu, J., Awadalla, P., Duan, J., McGee, K. M., Joy, D. A., McVean, G. A., and Su, X.-Z. (2005) PLoS Biol. 3 e335. [DOI] [PMC free article] [PubMed] [Google Scholar]
- 53.Cooper, R. A., Ferdig, M. T., Su, X.-Z., Ursos, L. M., Mu, J., Nomura, T., Fujioka, H., Fidock, D. A., Roepe, P. D., and Wellems, T. E. (2002) Mol. Pharmacol. 61 35-42 [DOI] [PubMed] [Google Scholar]
- 54.Wongsrichanalai, C., and Meshnick, S. R. (2008) Emerg. Infect. Dis. 14 716-719 [DOI] [PMC free article] [PubMed] [Google Scholar]
- 55.Ginsburg, H., and Golenser, J. (2003) Redox. Rep. 8 276-279 [DOI] [PubMed] [Google Scholar]
- 56.Kawazu, S., Komaki-Yasuda, K., Oku, H., and Kano, S. (2008) Parasitol. Int. 57 1-7 [DOI] [PubMed] [Google Scholar]
- 57.Borst, P., Evers, R., Kool, M., and Wijnholds, J. (2000) J. Natl. Cancer Inst. 92 1295-1302 [DOI] [PubMed] [Google Scholar]
- 58.Peklak-Scott, C., Townsend, A. J., and Morrow, C. S. (2005) Biochemistry (Mosc.) 44 4426-4433 [DOI] [PubMed] [Google Scholar]
- 59.Sanchez, C. P., Rotmann, A., Stein, W. D., and Lanzer, M. (2008) Mol. Microbiol. 70 786-798 [DOI] [PubMed] [Google Scholar]
- 60.Cowman, A. F., Karcz, S., Galatis, D., and Culvenor, J. G. (1991) J. Cell Biol. 113 1033-1042 [DOI] [PMC free article] [PubMed] [Google Scholar]
- 61.Foote, S. J., Kyle, D. E., Martin, R. K., Oduola, A. M., Forsyth, K., Kemp, D. J., and Cowman, A. F. (1990) Nature 345 255-258 [DOI] [PubMed] [Google Scholar]
Associated Data
This section collects any data citations, data availability statements, or supplementary materials included in this article.