Abstract
A clear prediction of the helix-coil model for force generation in muscle is that force should be produced when the equilibrium (helix-coil) of a rigor (or activated) fiber is perturbed by a temperature jump near the melting temperature of the light meromyosin/heavy meromyosin hinge. An infrared, iodine-photodissociation laser was used to heat the fibers by approximately equal to 5 degrees C in under 1 mus. Under ionic conditions where rigor bridges are predominantly associated with the thick filament backbone, an abrupt drop in tension typical of normal thermoelastic expansion was seen. A similar response was observed below 41 degrees C for thick filament-released rigor bridges. Above this temperature, a rubber-like thermoelastic response was obtained typical of a helix-coil transition. At temperatures near 50 degrees C, the amount of force generated by a rigor fiber was large and comparable to that seen for an activated fiber at 5 degrees C. The relaxation spectra of force generation obtained for both systems (rigor and activated) show a step change followed by a biexponential kinetic process. The reciprocal relaxation times and amplitudes for these individual processes in activated and rigor fibers differ only by factors of 2-4. Force generation in the rigor muscle appears to arise from melting in the subfragment 2 hinge region of the myosin molecule since binding of subfragment 2 to the thick filament backbone inhibits force production. No significant force generation was observed following temperature jumps of relaxed fibers.
Full text
PDF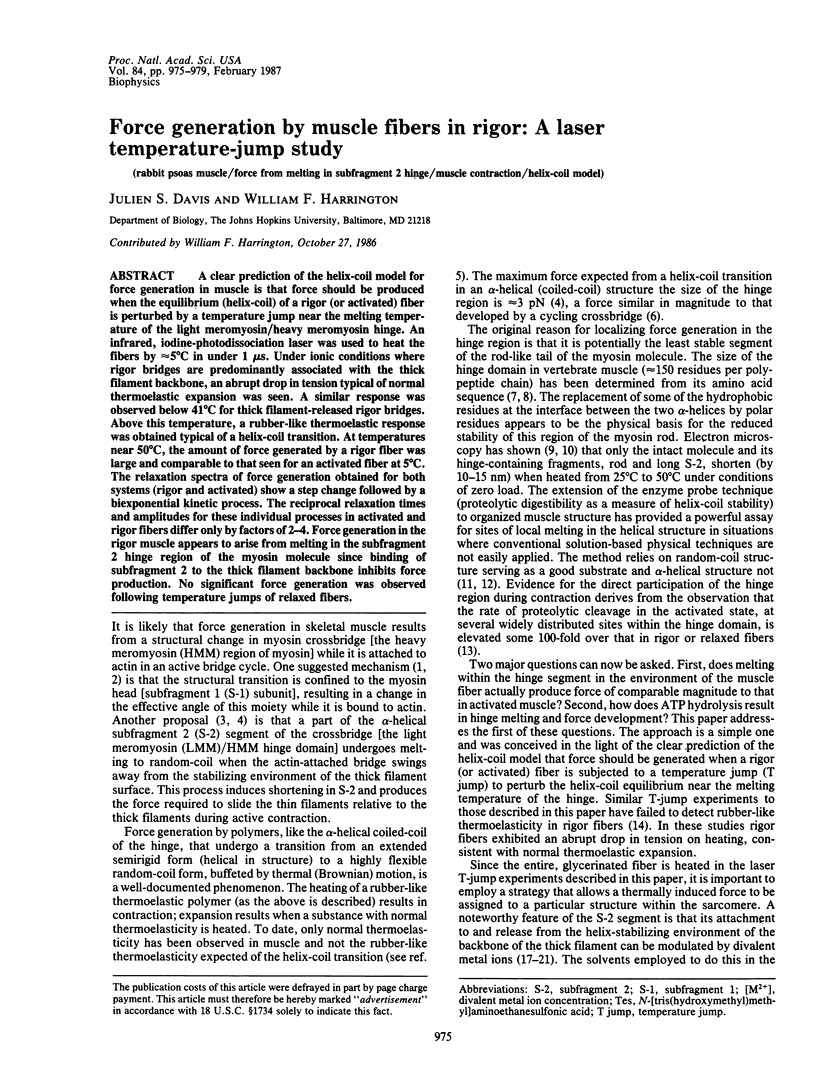
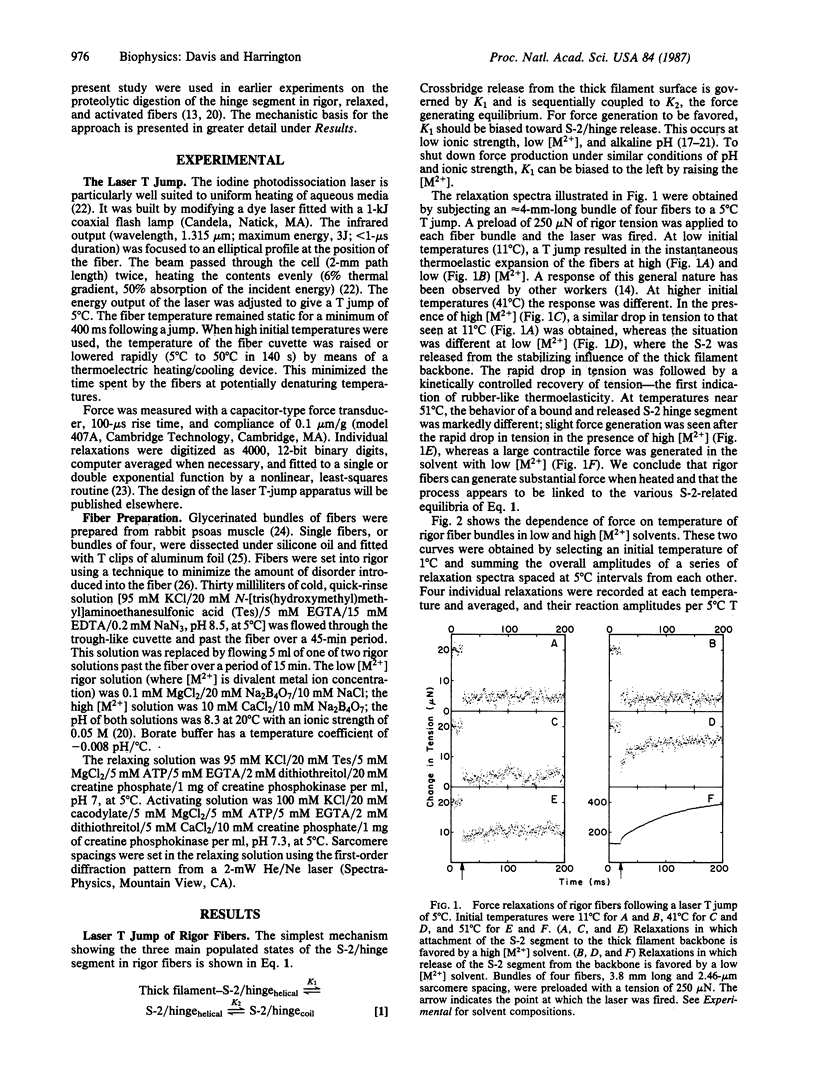
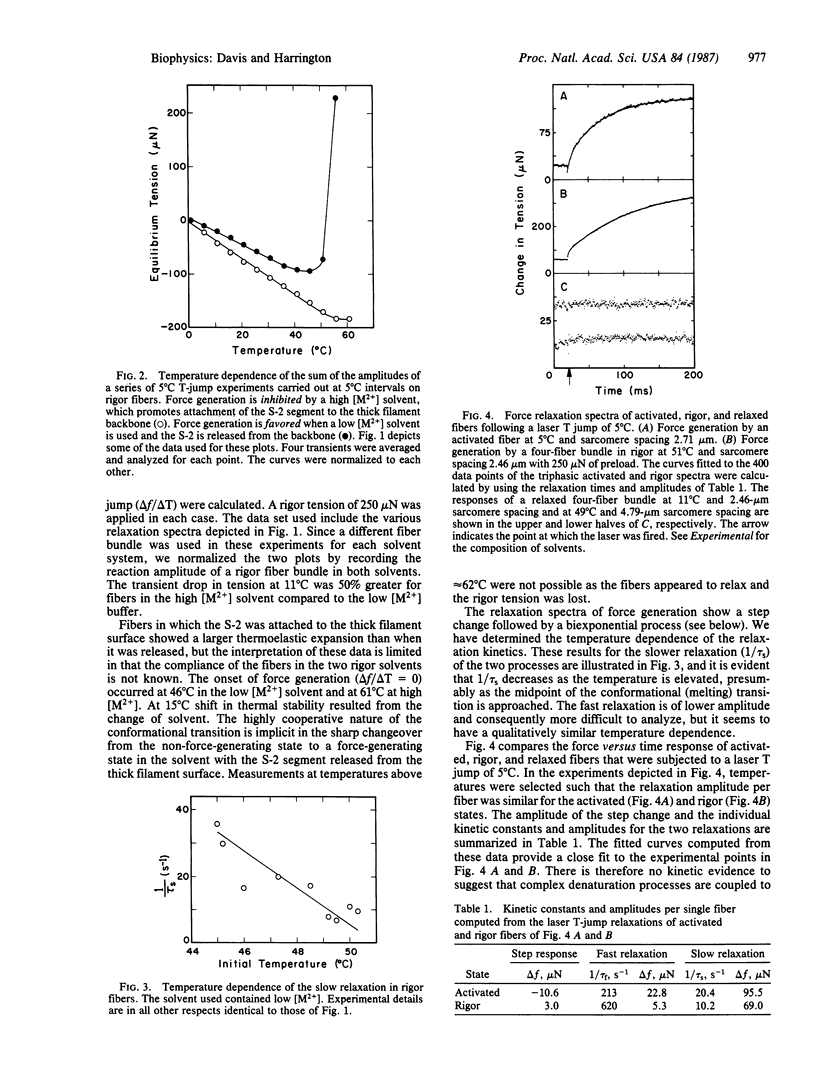
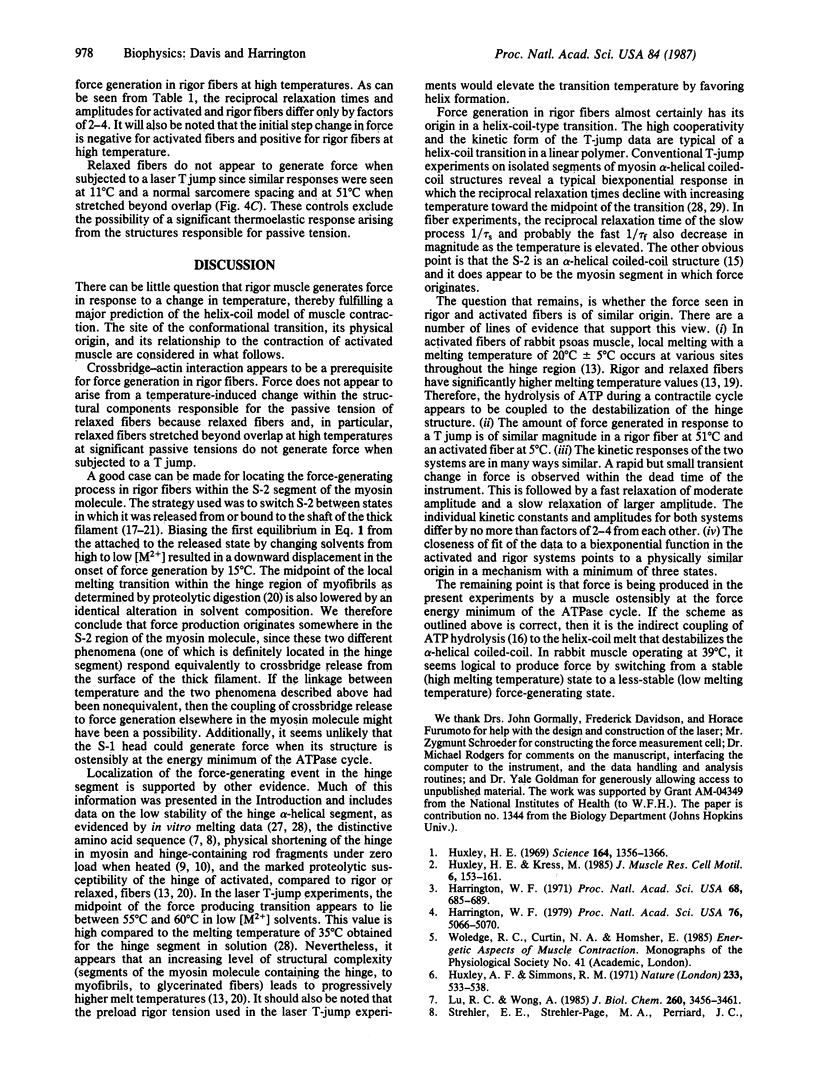
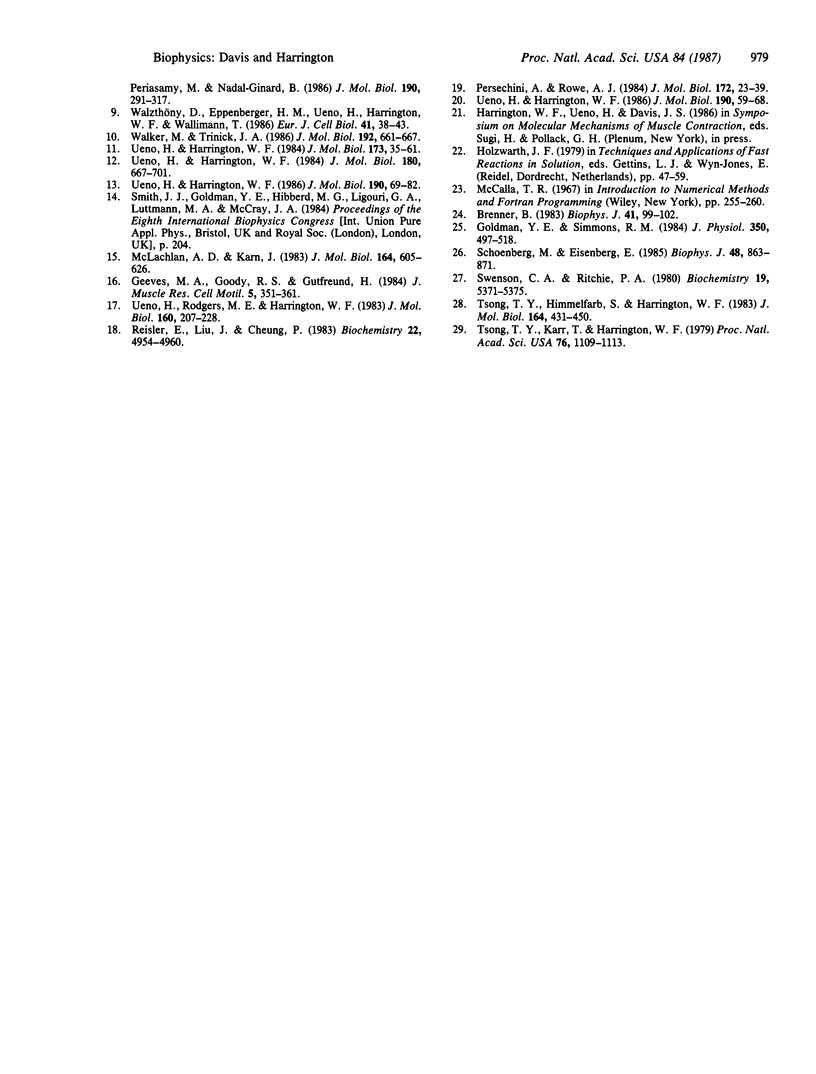
Selected References
These references are in PubMed. This may not be the complete list of references from this article.
- Brenner B. Technique for stabilizing the striation pattern in maximally calcium-activated skinned rabbit psoas fibers. Biophys J. 1983 Jan;41(1):99–102. doi: 10.1016/S0006-3495(83)84411-7. [DOI] [PMC free article] [PubMed] [Google Scholar]
- Geeves M. A., Goody R. S., Gutfreund H. Kinetics of acto-S1 interaction as a guide to a model for the crossbridge cycle. J Muscle Res Cell Motil. 1984 Aug;5(4):351–361. doi: 10.1007/BF00818255. [DOI] [PubMed] [Google Scholar]
- Goldman Y. E., Simmons R. M. Control of sarcomere length in skinned muscle fibres of Rana temporaria during mechanical transients. J Physiol. 1984 May;350:497–518. doi: 10.1113/jphysiol.1984.sp015215. [DOI] [PMC free article] [PubMed] [Google Scholar]
- Harrington W. F. A mechanochemical mechanism for muscle contraction. Proc Natl Acad Sci U S A. 1971 Mar;68(3):685–689. doi: 10.1073/pnas.68.3.685. [DOI] [PMC free article] [PubMed] [Google Scholar]
- Harrington W. F. On the origin of the contractile force in skeletal muscle. Proc Natl Acad Sci U S A. 1979 Oct;76(10):5066–5070. doi: 10.1073/pnas.76.10.5066. [DOI] [PMC free article] [PubMed] [Google Scholar]
- Huxley A. F., Simmons R. M. Proposed mechanism of force generation in striated muscle. Nature. 1971 Oct 22;233(5321):533–538. doi: 10.1038/233533a0. [DOI] [PubMed] [Google Scholar]
- Huxley H. E., Kress M. Crossbridge behaviour during muscle contraction. J Muscle Res Cell Motil. 1985 Apr;6(2):153–161. doi: 10.1007/BF00713057. [DOI] [PubMed] [Google Scholar]
- Huxley H. E. The mechanism of muscular contraction. Science. 1969 Jun 20;164(3886):1356–1365. doi: 10.1126/science.164.3886.1356. [DOI] [PubMed] [Google Scholar]
- Lu R. C., Wong A. The amino acid sequence and stability predictions of the hinge region in myosin subfragment 2. J Biol Chem. 1985 Mar 25;260(6):3456–3461. [PubMed] [Google Scholar]
- McLachlan A. D., Karn J. Periodic features in the amino acid sequence of nematode myosin rod. J Mol Biol. 1983 Mar 15;164(4):605–626. doi: 10.1016/0022-2836(83)90053-0. [DOI] [PubMed] [Google Scholar]
- Persechini A., Rowe A. J. Modulation of myosin filament conformation by physiological levels of divalent cation. J Mol Biol. 1984 Jan 5;172(1):23–39. doi: 10.1016/0022-2836(84)90412-1. [DOI] [PubMed] [Google Scholar]
- Reisler E., Liu J., Cheung P. Role of magnesium binding to myosin in controlling the state of cross-bridges in skeletal rabbit muscle. Biochemistry. 1983 Oct 11;22(21):4954–4960. doi: 10.1021/bi00290a012. [DOI] [PubMed] [Google Scholar]
- Schoenberg M., Eisenberg E. Muscle cross-bridge kinetics in rigor and in the presence of ATP analogues. Biophys J. 1985 Dec;48(6):863–871. doi: 10.1016/S0006-3495(85)83847-9. [DOI] [PMC free article] [PubMed] [Google Scholar]
- Strehler E. E., Strehler-Page M. A., Perriard J. C., Periasamy M., Nadal-Ginard B. Complete nucleotide and encoded amino acid sequence of a mammalian myosin heavy chain gene. Evidence against intron-dependent evolution of the rod. J Mol Biol. 1986 Aug 5;190(3):291–317. doi: 10.1016/0022-2836(86)90003-3. [DOI] [PubMed] [Google Scholar]
- Swenson C. A., Ritchie P. A. Conformational transitions in the subfragment-2 region of myosin. Biochemistry. 1980 Nov 11;19(23):5371–5375. doi: 10.1021/bi00564a035. [DOI] [PubMed] [Google Scholar]
- Tsong T. Y., Himmelfarb S., Harrington W. F. Stability and melting kinetics of structural domains in the myosin rod. J Mol Biol. 1983 Mar 5;164(3):431–450. doi: 10.1016/0022-2836(83)90060-8. [DOI] [PubMed] [Google Scholar]
- Tsong T. Y., Karr T., Harrington W. F. Rapid helix--coil transitions in the S-2 region of myosin. Proc Natl Acad Sci U S A. 1979 Mar;76(3):1109–1113. doi: 10.1073/pnas.76.3.1109. [DOI] [PMC free article] [PubMed] [Google Scholar]
- Ueno H., Harrington W. F. An enzyme-probe method to detect structural changes in the myosin rod. J Mol Biol. 1984 Feb 15;173(1):35–61. doi: 10.1016/0022-2836(84)90402-9. [DOI] [PubMed] [Google Scholar]
- Ueno H., Harrington W. F. An enzyme-probe study of motile domains in the subfragment-2 region of myosin. J Mol Biol. 1984 Dec 15;180(3):667–701. doi: 10.1016/0022-2836(84)90032-9. [DOI] [PubMed] [Google Scholar]
- Ueno H., Harrington W. F. Local melting in the subfragment-2 region of myosin in activated muscle and its correlation with contractile force. J Mol Biol. 1986 Jul 5;190(1):69–82. doi: 10.1016/0022-2836(86)90076-8. [DOI] [PubMed] [Google Scholar]
- Ueno H., Harrington W. F. Temperature-dependence of local melting in the myosin subfragment-2 region of the rigor cross-bridge. J Mol Biol. 1986 Jul 5;190(1):59–68. doi: 10.1016/0022-2836(86)90075-6. [DOI] [PubMed] [Google Scholar]
- Ueno H., Rodgers M. E., Harrington W. F. Self-association of a high molecular weight subfragment-2 of myosin induced by divalent metal ions. J Mol Biol. 1983 Aug 5;168(2):207–228. doi: 10.1016/s0022-2836(83)80015-1. [DOI] [PubMed] [Google Scholar]
- Walker M., Trinick J. Electron microscope study of the effect of temperature on the length of the tail of the myosin molecule. J Mol Biol. 1986 Dec 5;192(3):661–667. doi: 10.1016/0022-2836(86)90283-4. [DOI] [PubMed] [Google Scholar]
- Walzthöny D., Eppenberger H. M., Ueno H., Harrington W. F., Wallimann T. Melting of myosin rod as revealed by electron microscopy. II. Effects of temperature and pH on length and stability of myosin rod and its fragments. Eur J Cell Biol. 1986 Jun;41(1):38–43. [PubMed] [Google Scholar]