Abstract
Three types of metabolic control of oxidative metabolism are observed in the various tissues that have been studied by phosphorous magnetic resonance spectroscopy. The principal control of oxidative metabolism in skeletal muscle is by ADP (or Pi/phosphocreatine). This conclusion is based upon studies of arm muscles of humans during steady-state exercise. A work-cost (Vm vs. Pi/phosphocreatine) relationship follows a Michaelis-Menten rectangular hyperbola, where Km values from 0.5 to 0.6 and Vmax values from 50 to 200 (at nearly constant pH) are found in linearized plots of the equation V/Vmax = 1/(1 + 0.6 phosphocreatine/Pi) where V is work level (which is equal to the velocity of the enzymatic reaction) and Vmax is the maximal work capacity that is a measure of the enzyme activity (E) of oxidative metabolism. Adaptation to exercise enhances the slope of the work-cost relationship and causes large changes in Vmax or E. A second metabolic control may enhance the slope of the work-cost relationship but not Vmax. For example, the initiation of exercise can lead to an improved characteristic that can be explained by 2-fold increased substrate delivery, for example, increased oxygen delivery by microcirculatory control. Cardiac tissue of the adult dog affords an example of optimal endurance performance adaptation and exhibits the steepest work-cost relationship observed and is attributed to a coordinated control of substrate delivery that may involve Ca2+ and inorganic phosphate control of NADH; control of O2 delivery may also be involved. The calculated work-cost relationship is similar to that observed in the beagle heart. The theoretical curve illustrates that the liability of multiple controls is a sharp break point in metabolic control at the end of the multiple control range--a possible cause of instability of cardiac performance at high V/Vmax.
Full text
PDF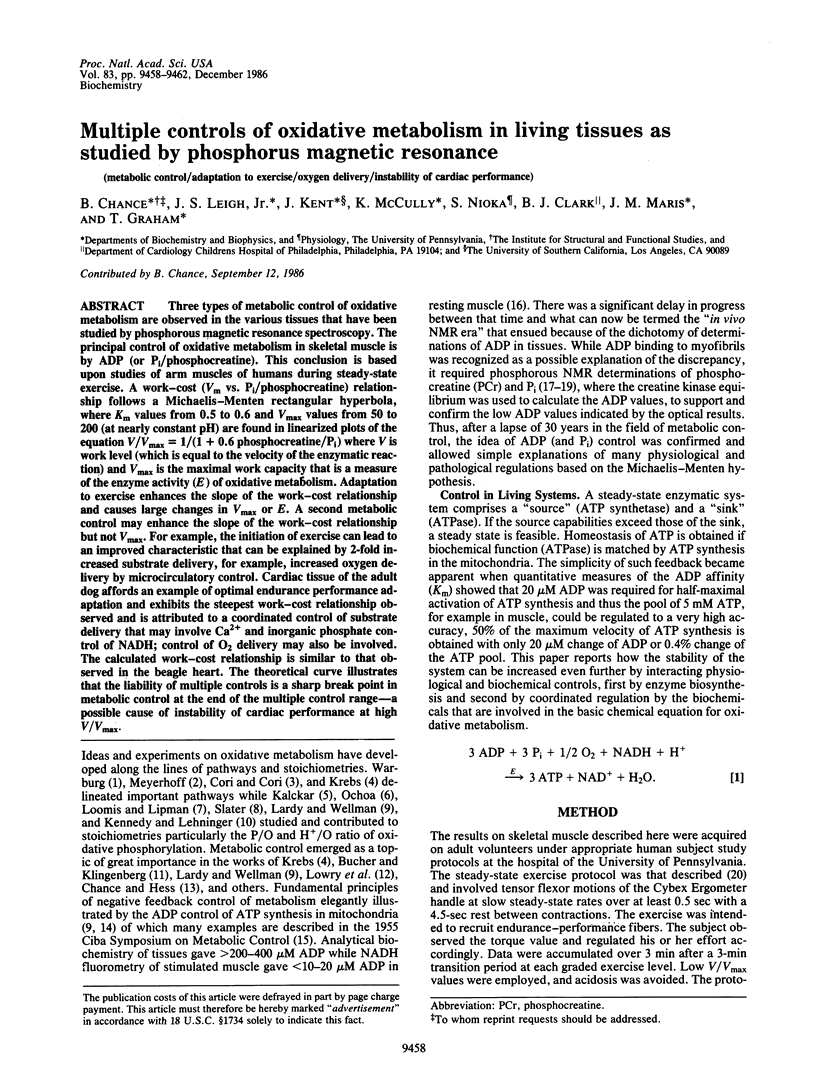
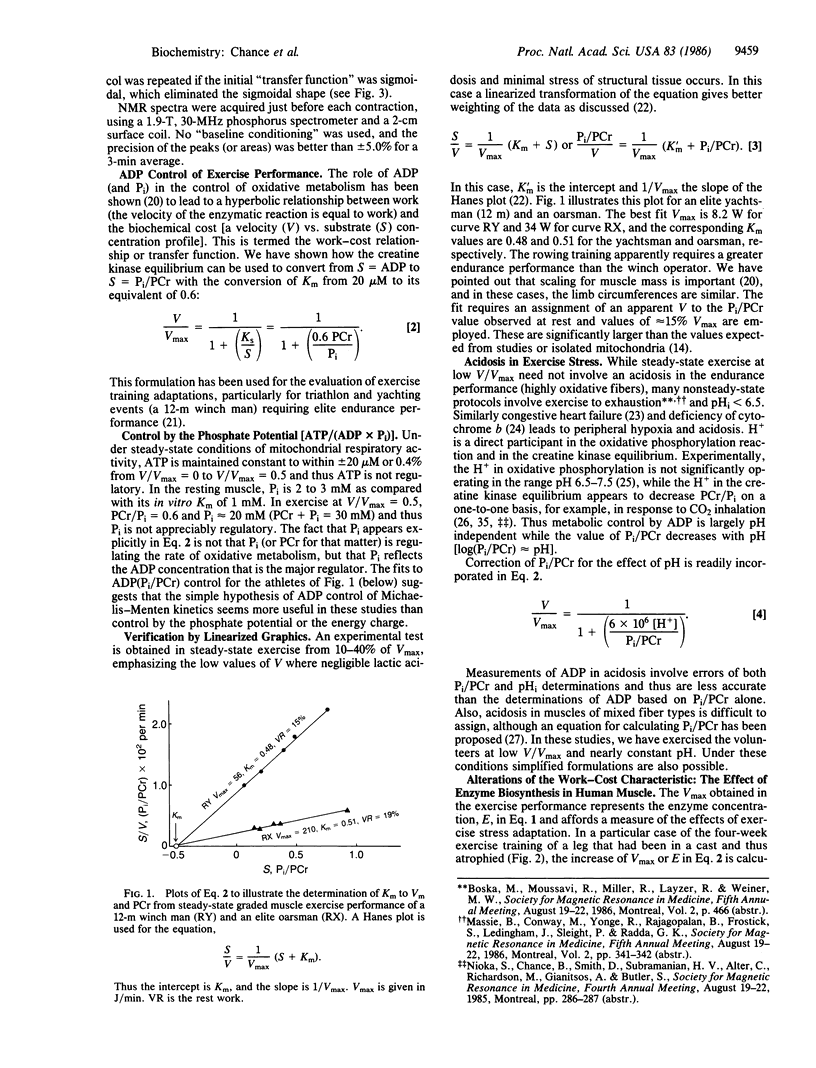
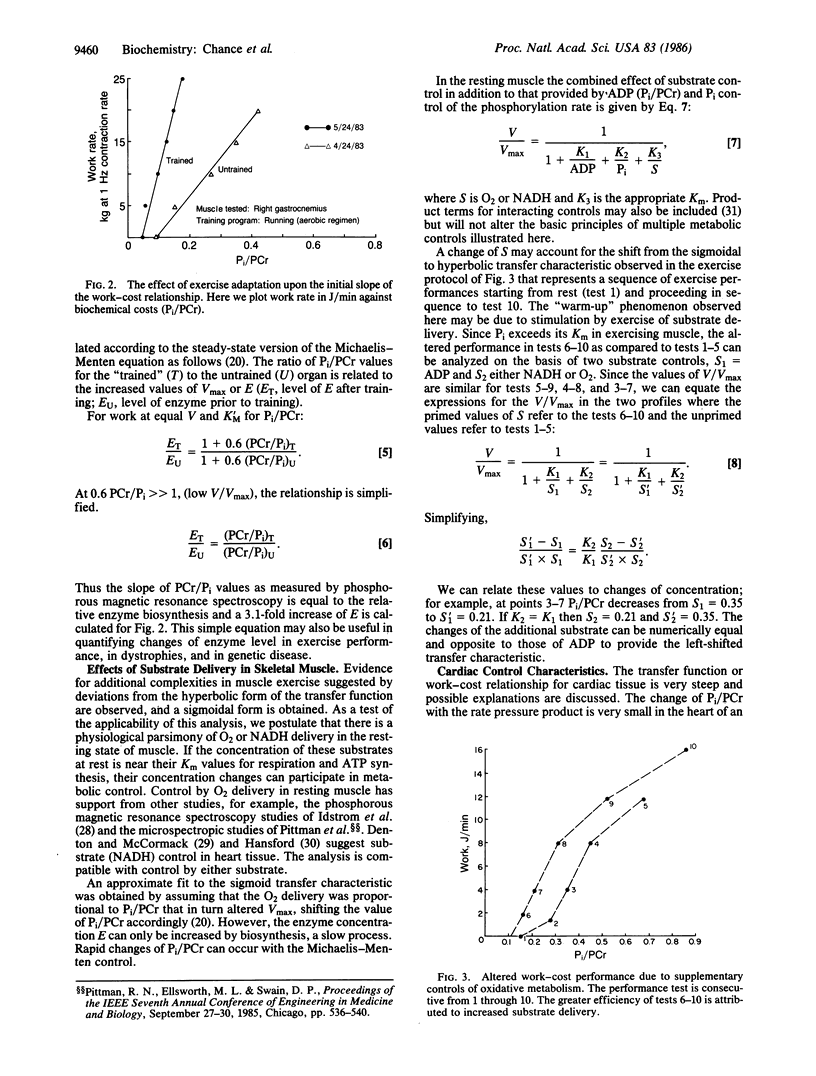
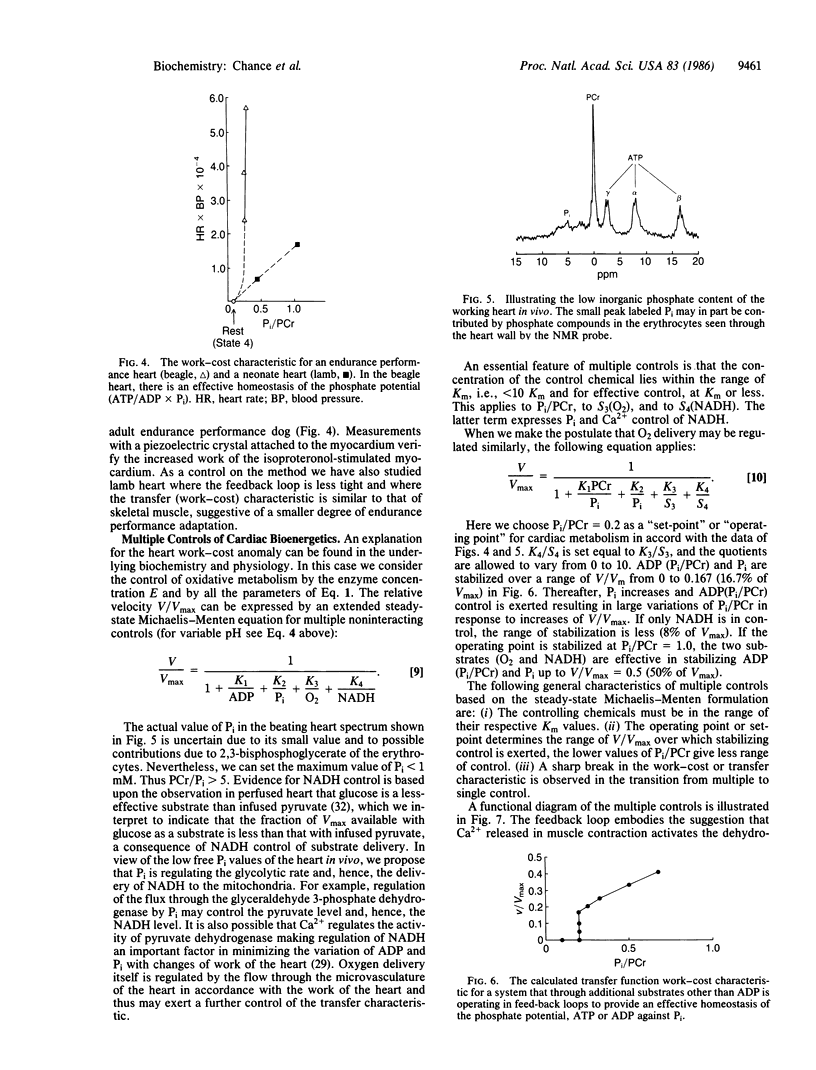
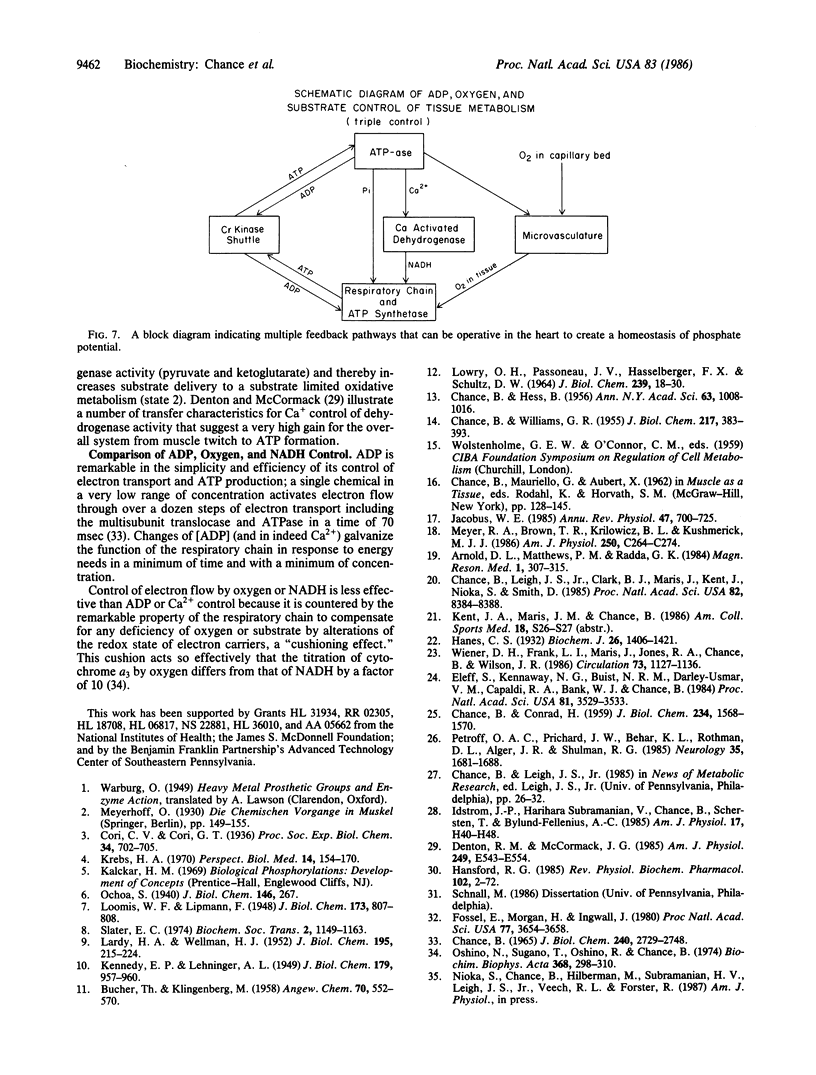
Selected References
These references are in PubMed. This may not be the complete list of references from this article.
- Arnold D. L., Matthews P. M., Radda G. K. Metabolic recovery after exercise and the assessment of mitochondrial function in vivo in human skeletal muscle by means of 31P NMR. Magn Reson Med. 1984 Sep;1(3):307–315. doi: 10.1002/mrm.1910010303. [DOI] [PubMed] [Google Scholar]
- CHANCE B., CONRAD H. Acid-linked functions of intermediates in oxidative phosphorylation. II. Experimental studies of the effect of pH upon respiratory, phosphorylative and transfer activities of liver and heart mitochondria. J Biol Chem. 1959 Jun;234(6):1568–1570. [PubMed] [Google Scholar]
- CHANCE B., HESS B. On the control of metabolism in ascites tumor cell suspensions. Ann N Y Acad Sci. 1956 Mar 14;63(5):1008–1016. doi: 10.1111/j.1749-6632.1956.tb50908.x. [DOI] [PubMed] [Google Scholar]
- CHANCE B. THE ENERGY-LINKED REACTION OF CALCIUM WITH MITOCHONDRIA. J Biol Chem. 1965 Jun;240:2729–2748. [PubMed] [Google Scholar]
- CHANCE B., WILLIAMS G. R. Respiratory enzymes in oxidative phosphorylation. I. Kinetics of oxygen utilization. J Biol Chem. 1955 Nov;217(1):383–393. [PubMed] [Google Scholar]
- Chance B., Leigh J. S., Jr, Clark B. J., Maris J., Kent J., Nioka S., Smith D. Control of oxidative metabolism and oxygen delivery in human skeletal muscle: a steady-state analysis of the work/energy cost transfer function. Proc Natl Acad Sci U S A. 1985 Dec;82(24):8384–8388. doi: 10.1073/pnas.82.24.8384. [DOI] [PMC free article] [PubMed] [Google Scholar]
- Denton R. M., McCormack J. G. Ca2+ transport by mammalian mitochondria and its role in hormone action. Am J Physiol. 1985 Dec;249(6 Pt 1):E543–E554. doi: 10.1152/ajpendo.1985.249.6.E543. [DOI] [PubMed] [Google Scholar]
- Eleff S., Kennaway N. G., Buist N. R., Darley-Usmar V. M., Capaldi R. A., Bank W. J., Chance B. 31P NMR study of improvement in oxidative phosphorylation by vitamins K3 and C in a patient with a defect in electron transport at complex III in skeletal muscle. Proc Natl Acad Sci U S A. 1984 Jun;81(11):3529–3533. doi: 10.1073/pnas.81.11.3529. [DOI] [PMC free article] [PubMed] [Google Scholar]
- Fossel E. T., Morgan H. E., Ingwall J. S. Measurement of changes in high-energy phosphates in the cardiac cycle using gated 31P nuclear magnetic renonance. Proc Natl Acad Sci U S A. 1980 Jun;77(6):3654–3658. doi: 10.1073/pnas.77.6.3654. [DOI] [PMC free article] [PubMed] [Google Scholar]
- Hanes C. S. Studies on plant amylases: The effect of starch concentration upon the velocity of hydrolysis by the amylase of germinated barley. Biochem J. 1932;26(5):1406–1421. doi: 10.1042/bj0261406. [DOI] [PMC free article] [PubMed] [Google Scholar]
- Hansford R. G. Relation between mitochondrial calcium transport and control of energy metabolism. Rev Physiol Biochem Pharmacol. 1985;102:1–72. doi: 10.1007/BFb0034084. [DOI] [PubMed] [Google Scholar]
- Idström J. P., Subramanian V. H., Chance B., Schersten T., Bylund-Fellenius A. C. Oxygen dependence of energy metabolism in contracting and recovering rat skeletal muscle. Am J Physiol. 1985 Jan;248(1 Pt 2):H40–H48. doi: 10.1152/ajpheart.1985.248.1.H40. [DOI] [PubMed] [Google Scholar]
- Jacobus W. E. Respiratory control and the integration of heart high-energy phosphate metabolism by mitochondrial creatine kinase. Annu Rev Physiol. 1985;47:707–725. doi: 10.1146/annurev.ph.47.030185.003423. [DOI] [PubMed] [Google Scholar]
- Krebs H. A. The history of the tricarboxylic acid cycle. Perspect Biol Med. 1970 Autumn;14(1):154–170. doi: 10.1353/pbm.1970.0001. [DOI] [PubMed] [Google Scholar]
- LARDY H. A., WELLMAN H. Oxidative phosphorylations; rôle of inorganic phosphate and acceptor systems in control of metabolic rates. J Biol Chem. 1952 Mar;195(1):215–224. [PubMed] [Google Scholar]
- LOWRY O. H., PASSONNEAU J. V., HASSELBERGER F. X., SCHULZ D. W. EFFECT OF ISCHEMIA ON KNOWN SUBSTRATES AND COFACTORS OF THE GLYCOLYTIC PATHWAY IN BRAIN. J Biol Chem. 1964 Jan;239:18–30. [PubMed] [Google Scholar]
- Meyer R. A., Brown T. R., Krilowicz B. L., Kushmerick M. J. Phosphagen and intracellular pH changes during contraction of creatine-depleted rat muscle. Am J Physiol. 1986 Feb;250(2 Pt 1):C264–C274. doi: 10.1152/ajpcell.1986.250.2.C264. [DOI] [PubMed] [Google Scholar]
- Oshino N., Sugano T., Oshino R., Chance B. Mitochondrial function under hypoxic conditions: the steady states of cytochrome alpha+alpha3 and their relation to mitochondrial energy states. Biochim Biophys Acta. 1974 Dec 19;368(3):298–310. doi: 10.1016/0005-2728(74)90176-5. [DOI] [PubMed] [Google Scholar]
- Petroff O. A., Prichard J. W., Behar K. L., Rothman D. L., Alger J. R., Shulman R. G. Cerebral metabolism in hyper- and hypocarbia: 31P and 1H nuclear magnetic resonance studies. Neurology. 1985 Dec;35(12):1681–1688. doi: 10.1212/wnl.35.12.1681. [DOI] [PubMed] [Google Scholar]
- Wiener D. H., Fink L. I., Maris J., Jones R. A., Chance B., Wilson J. R. Abnormal skeletal muscle bioenergetics during exercise in patients with heart failure: role of reduced muscle blood flow. Circulation. 1986 Jun;73(6):1127–1136. doi: 10.1161/01.cir.73.6.1127. [DOI] [PubMed] [Google Scholar]