Abstract
1. The fully activated current-voltage relation (I-V) of the delayed rectifier potassium ion channel in squid giant axons has a non-linear dependence upon the driving force, V-EK, as I have previously demonstrated, where V is membrane potential and EK is the equilibrium potential for potassium ions. 2. The non-linearity of the I-V relation and its dependence upon external potassium ion concentration are both well described, phenomenologically, by the Goldman-Hodgkin-Katz (GHK) flux equation, as I have also previously demonstrated. As illustrated below, this result can be modelled using the Eyring rate theory of single-file diffusion of ions through a channel in the low-occupancy limit of the theory. 3. The GHK equation analysis and the low-occupancy limit of the Eyring rate theory are both consistent with the independence principle for movement of ions through the channel, which is at odds with tracer flux ratio results from the delayed rectifier, published elsewhere. Those results suggest that the channel is multiply occupied by two, or perhaps three, ions. 4. The resolution of this paradox is provided by a triple-binding site, multiple-occupancy model in which only one vacancy, at most, is allowed in the channel. This model predicts current-voltage relations which are consistent with the data (and with the phenomenological prediction of the GHK flux equation). The model is also consistent, approximately, with the tracer flux ratio results.
Full text
PDF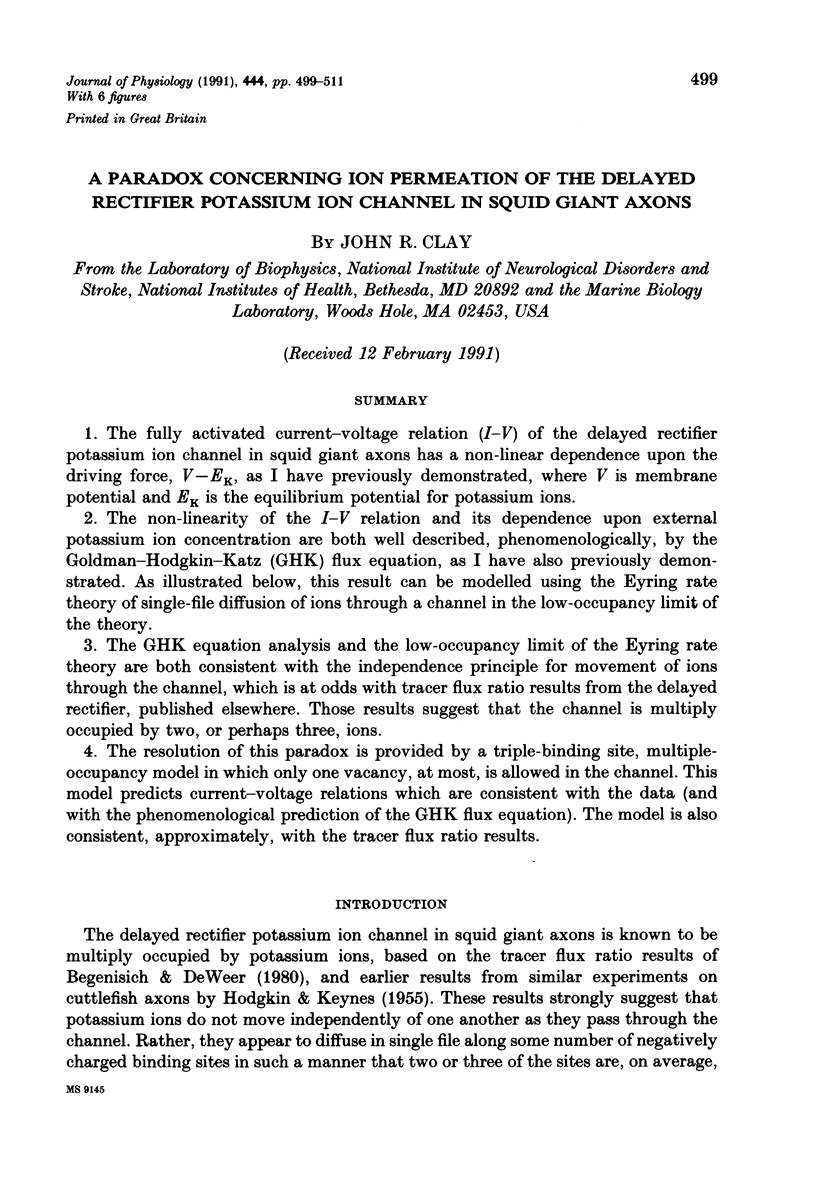
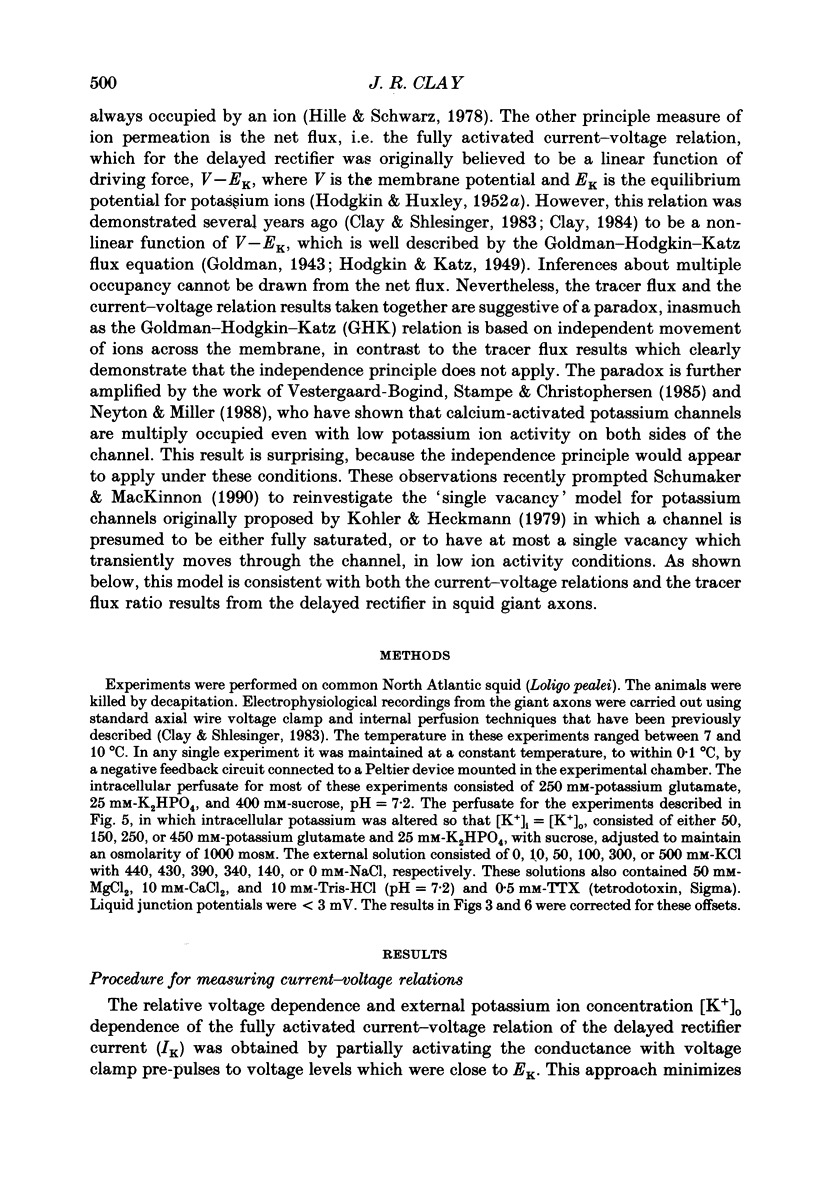
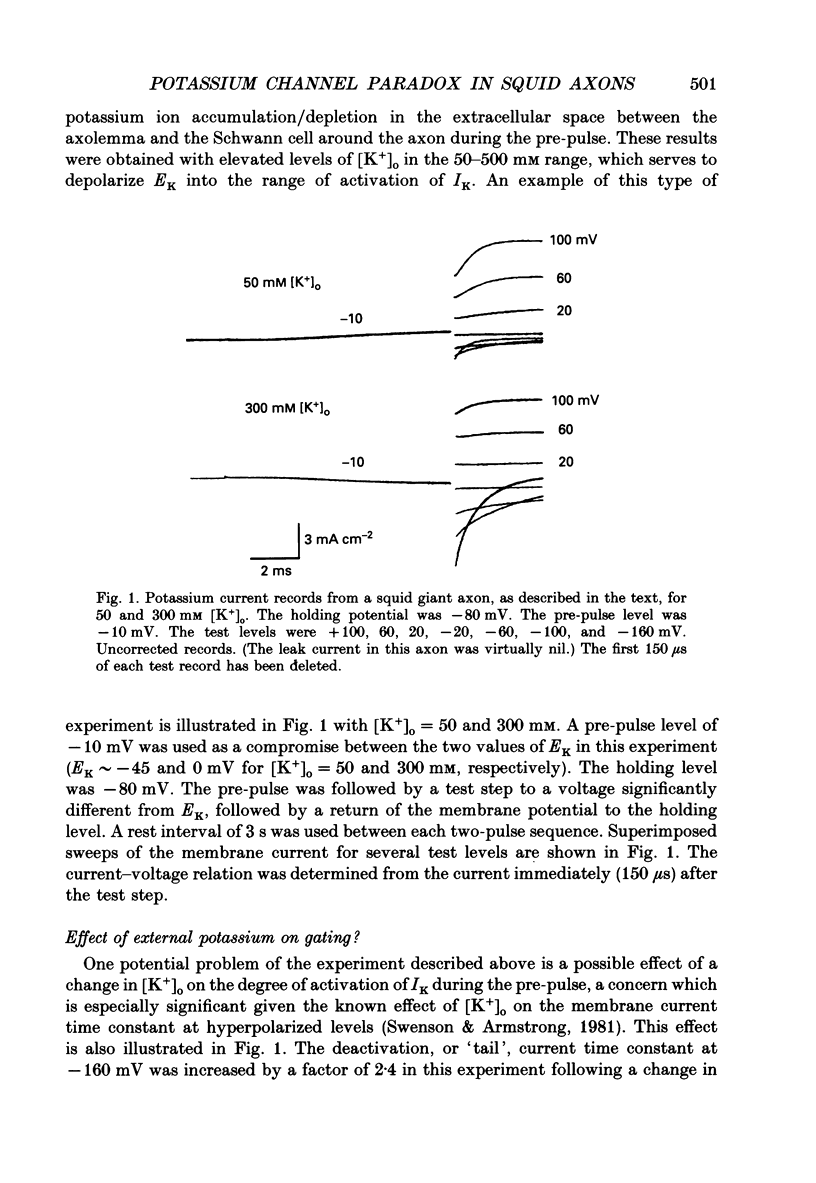
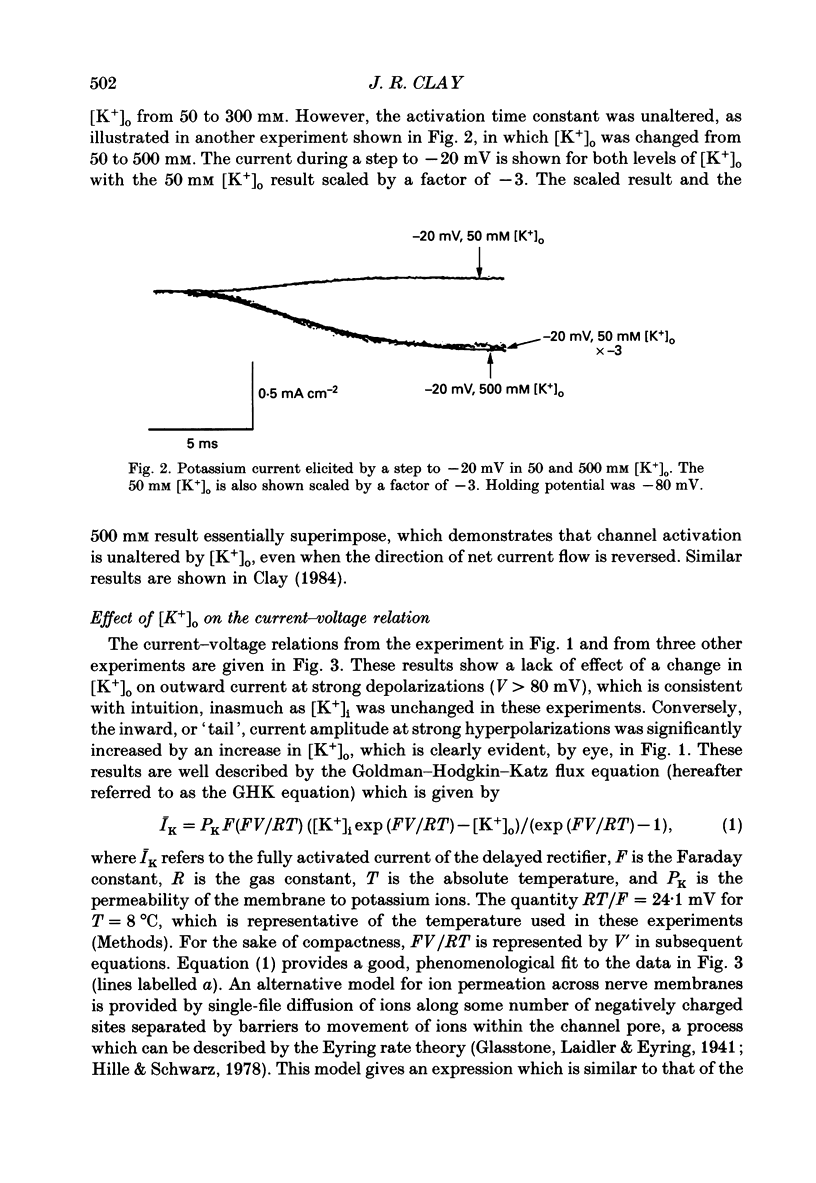
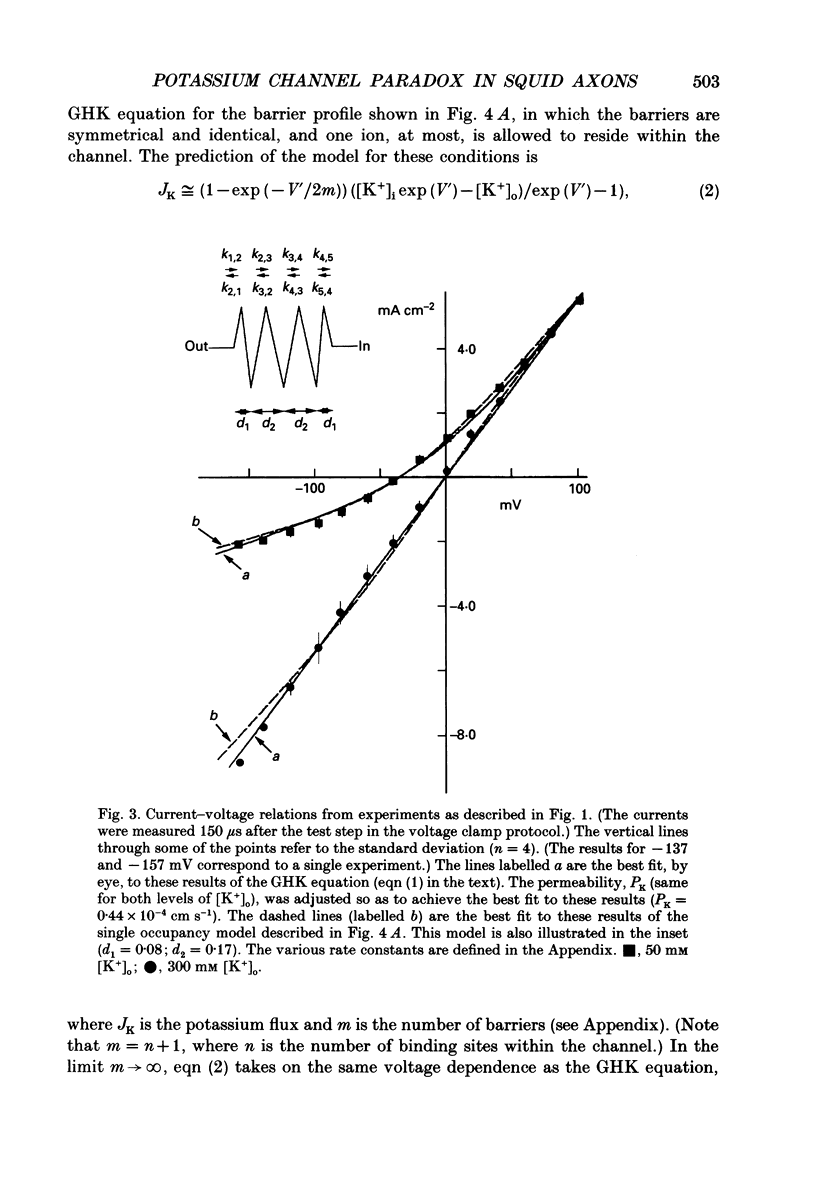
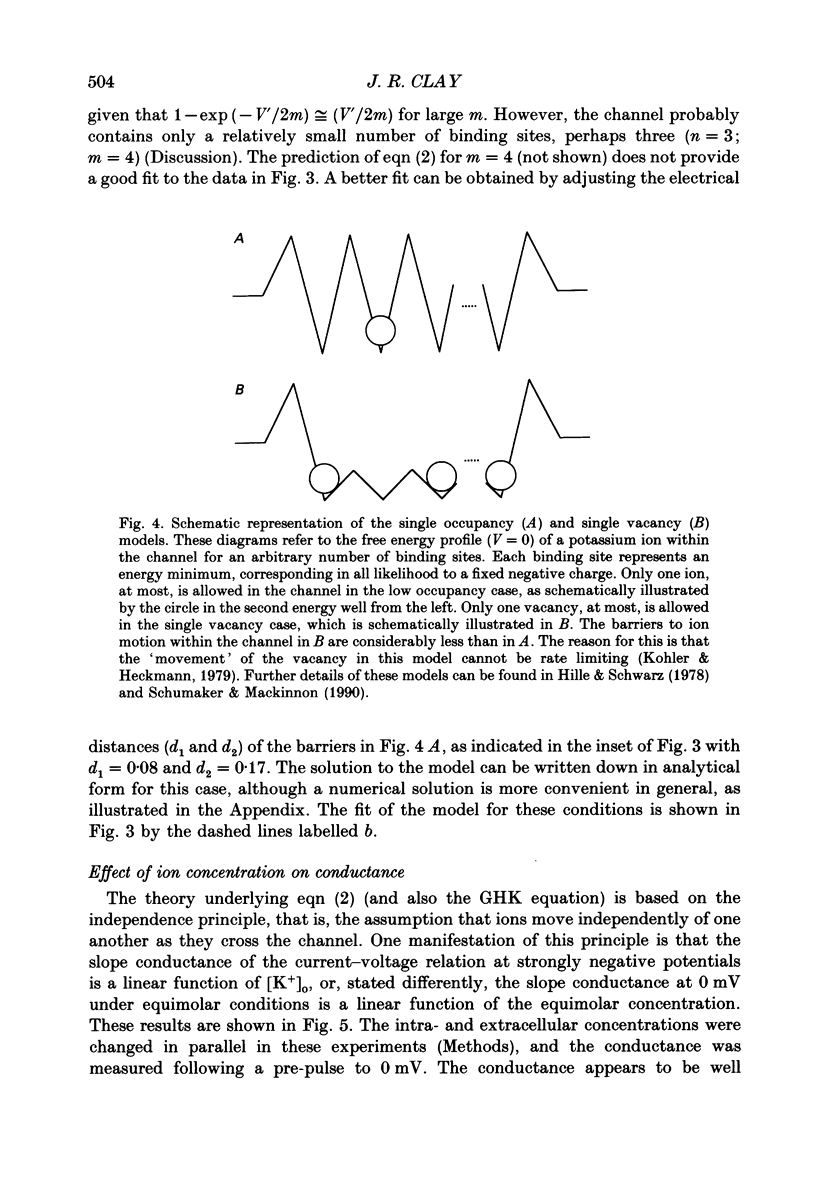
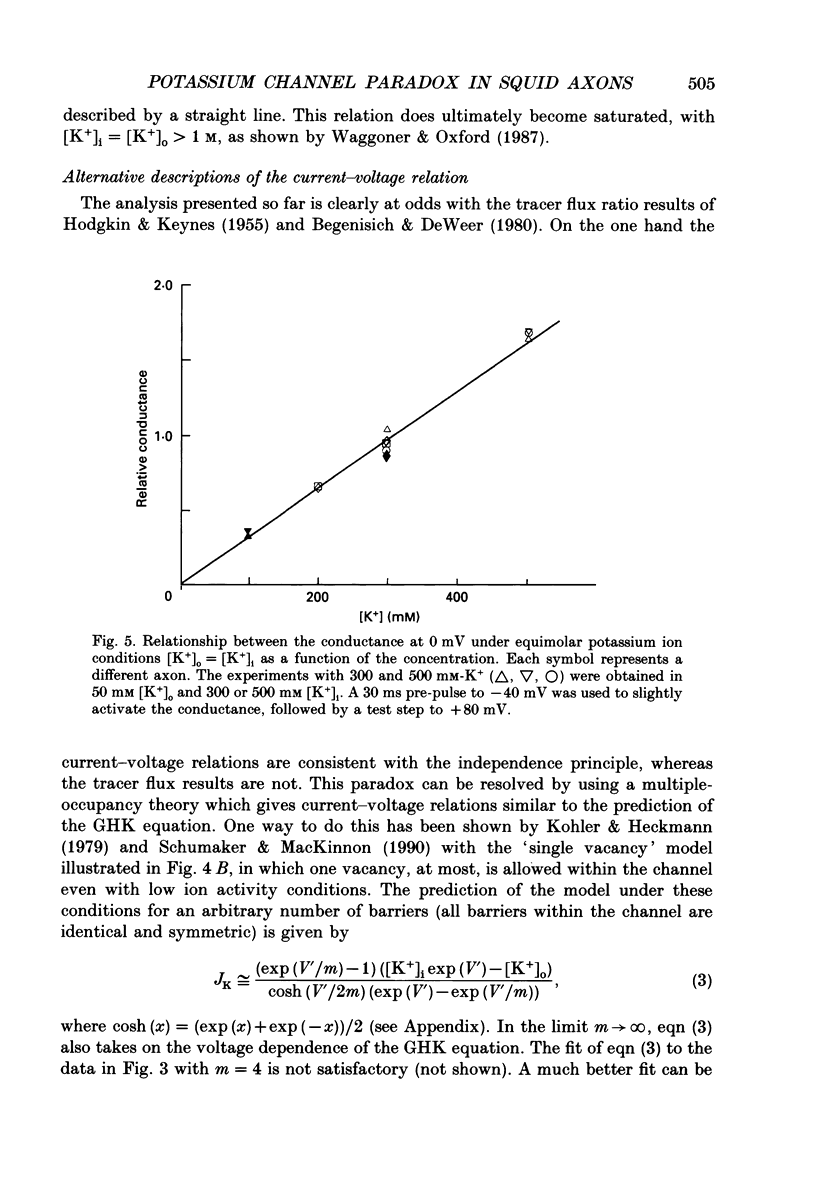
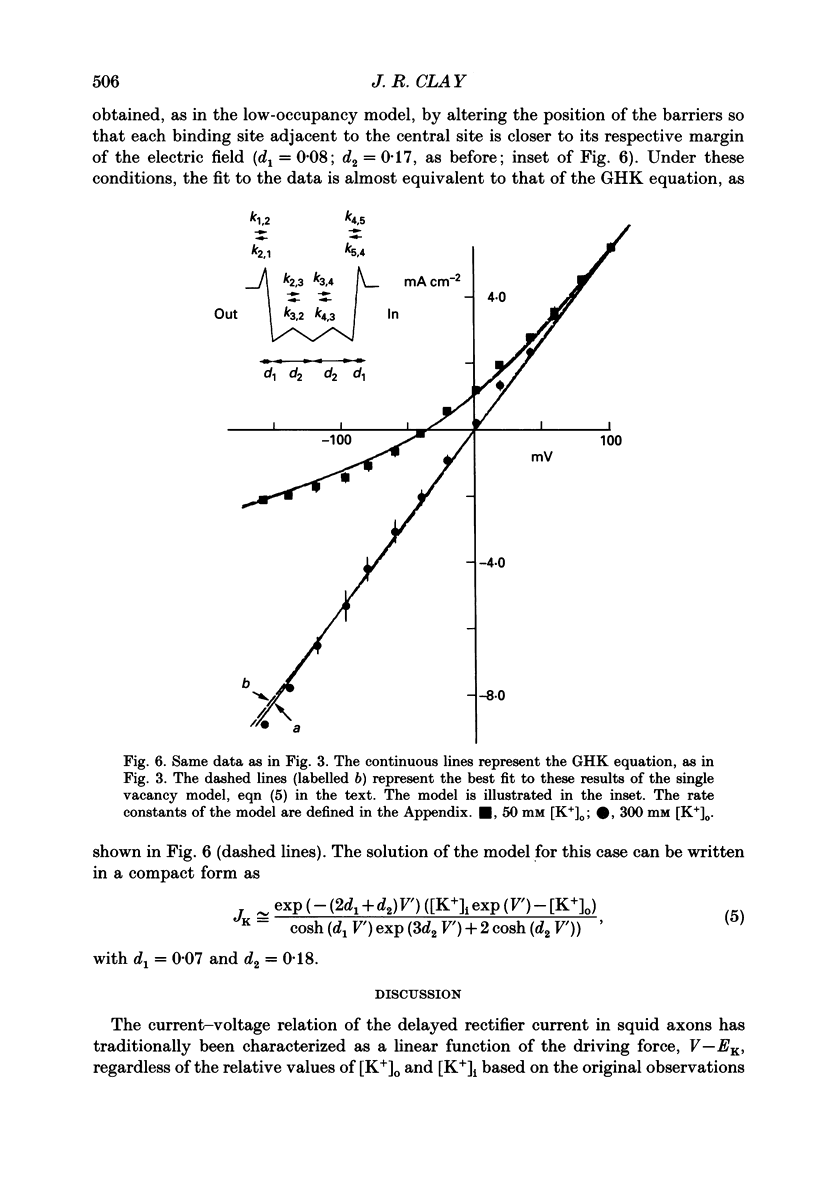
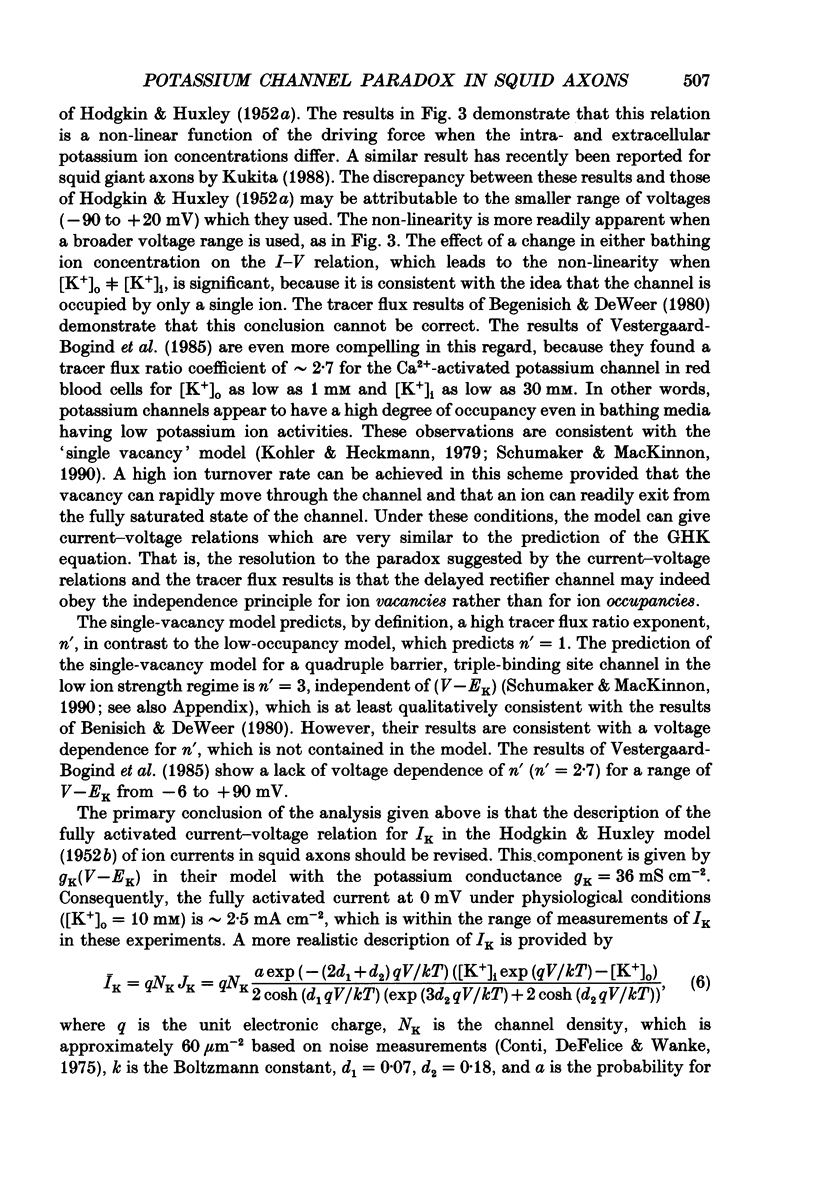
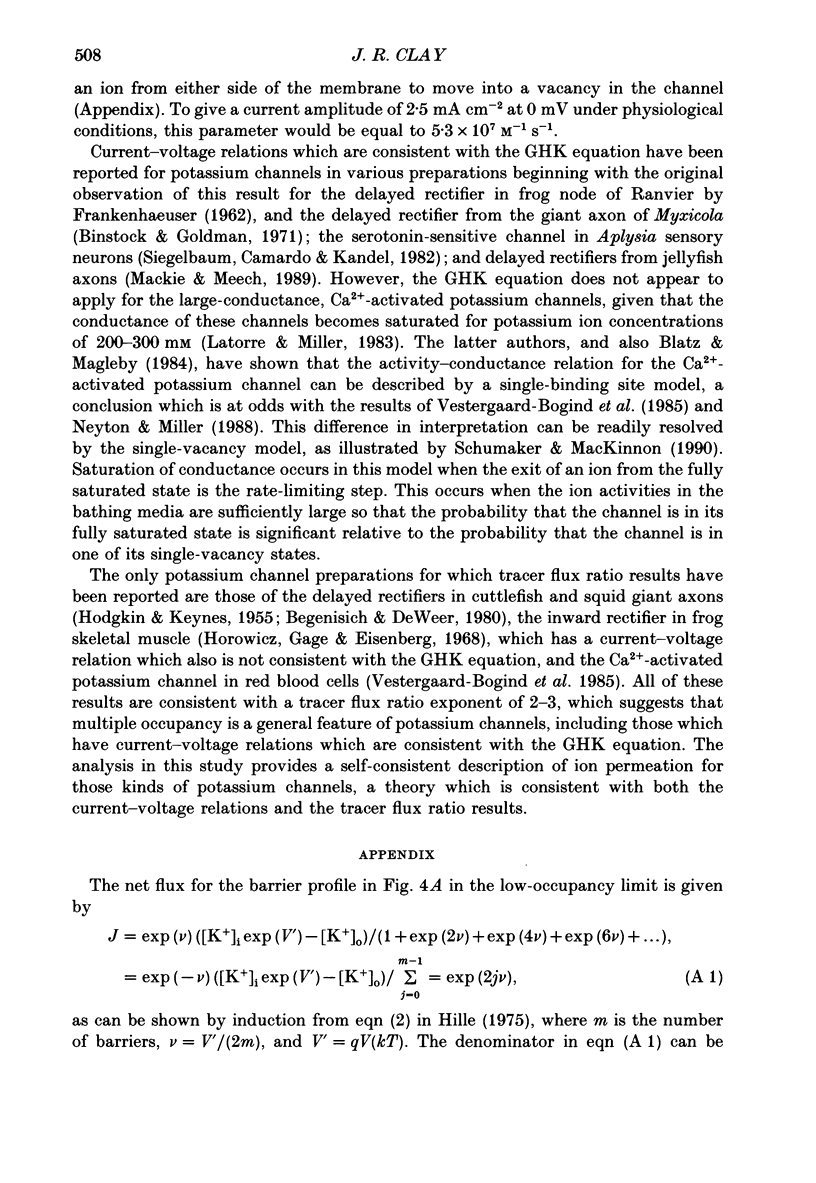
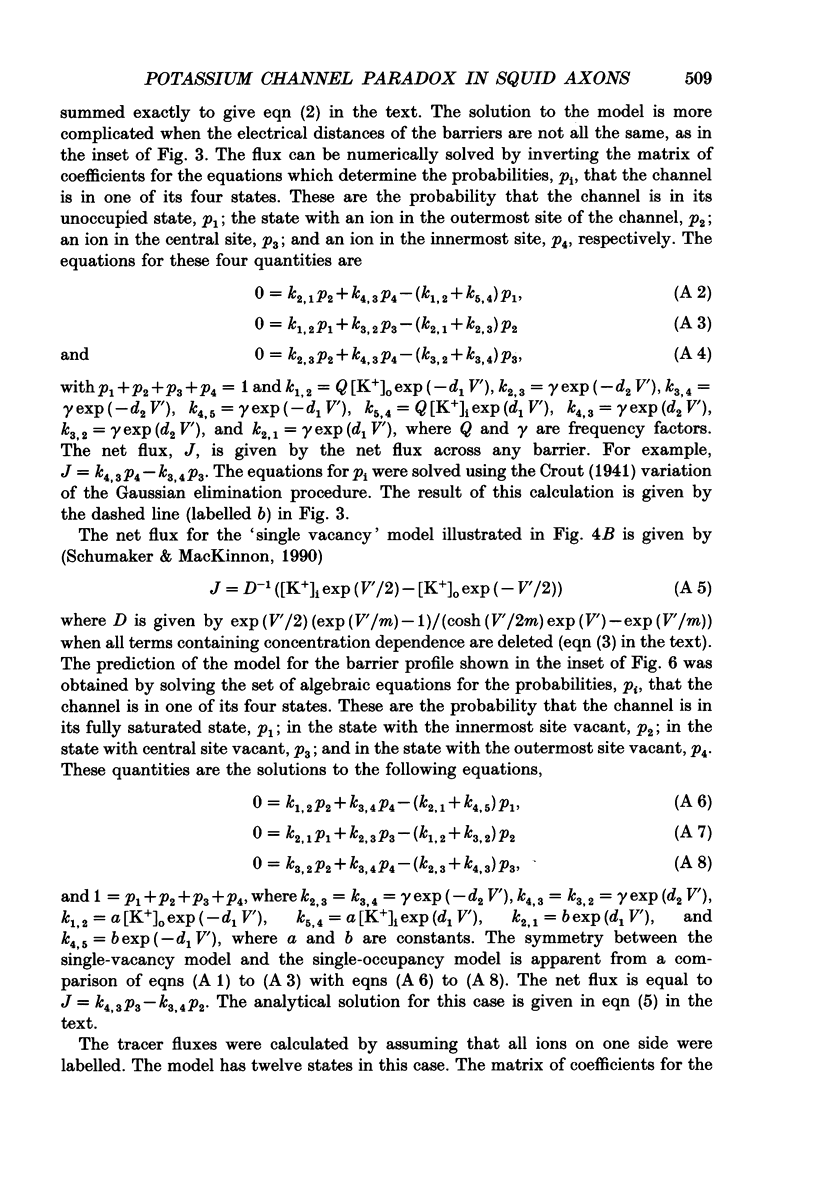
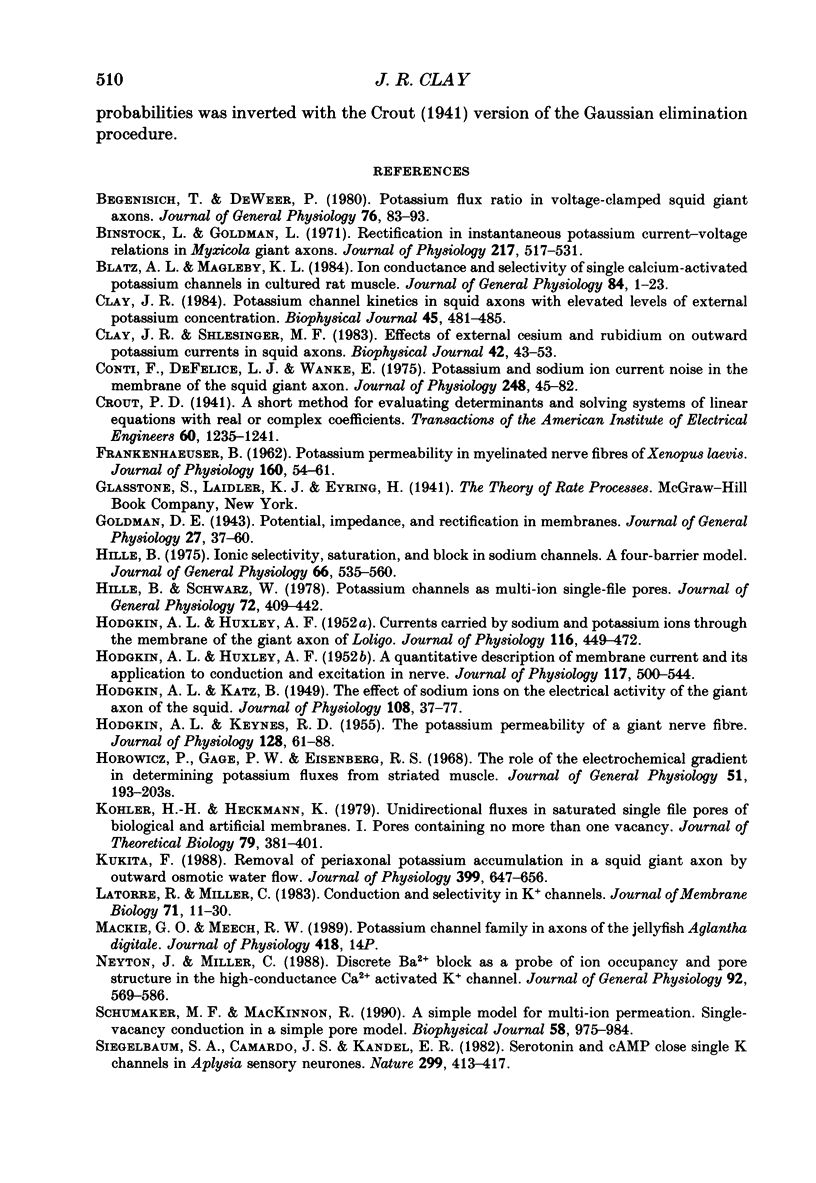
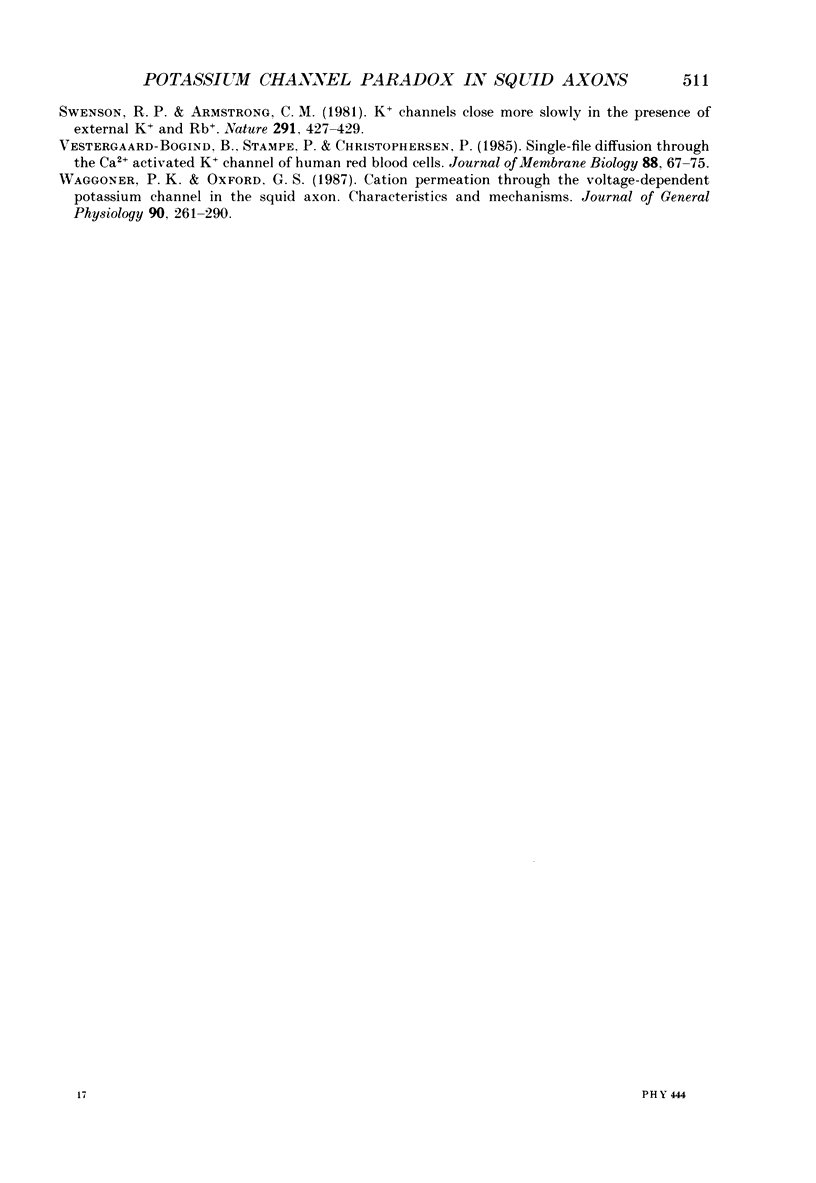
Selected References
These references are in PubMed. This may not be the complete list of references from this article.
- Begenisich T., De Weer P. Potassium flux ratio in voltage-clamped squid giant axons. J Gen Physiol. 1980 Jul;76(1):83–98. doi: 10.1085/jgp.76.1.83. [DOI] [PMC free article] [PubMed] [Google Scholar]
- Binstock L., Goldman L. Rectification in instantaneous potassium current-voltage relations in Myxicola giant axons. J Physiol. 1971 Sep;217(3):517–531. doi: 10.1113/jphysiol.1971.sp009583. [DOI] [PMC free article] [PubMed] [Google Scholar]
- Blatz A. L., Magleby K. L. Ion conductance and selectivity of single calcium-activated potassium channels in cultured rat muscle. J Gen Physiol. 1984 Jul;84(1):1–23. doi: 10.1085/jgp.84.1.1. [DOI] [PMC free article] [PubMed] [Google Scholar]
- Clay J. R. Potassium channel kinetics in squid axons with elevated levels of external potassium concentration. Biophys J. 1984 Feb;45(2):481–485. doi: 10.1016/S0006-3495(84)84172-7. [DOI] [PMC free article] [PubMed] [Google Scholar]
- Clay J. R., Shlesinger M. F. Effects of external cesium and rubidium on outward potassium currents in squid axons. Biophys J. 1983 Apr;42(1):43–53. doi: 10.1016/S0006-3495(83)84367-7. [DOI] [PMC free article] [PubMed] [Google Scholar]
- Conti F., De Felice L. J., Wanke E. Potassium and sodium ion current noise in the membrane of the squid giant axon. J Physiol. 1975 Jun;248(1):45–82. doi: 10.1113/jphysiol.1975.sp010962. [DOI] [PMC free article] [PubMed] [Google Scholar]
- FRANKENHAEUSER B. Potassium permeability in myelinated nerve fibres of Xenopus laevis. J Physiol. 1962 Jan;160:54–61. doi: 10.1113/jphysiol.1962.sp006834. [DOI] [PMC free article] [PubMed] [Google Scholar]
- Goldman D. E. POTENTIAL, IMPEDANCE, AND RECTIFICATION IN MEMBRANES. J Gen Physiol. 1943 Sep 20;27(1):37–60. doi: 10.1085/jgp.27.1.37. [DOI] [PMC free article] [PubMed] [Google Scholar]
- HODGKIN A. L., HUXLEY A. F. A quantitative description of membrane current and its application to conduction and excitation in nerve. J Physiol. 1952 Aug;117(4):500–544. doi: 10.1113/jphysiol.1952.sp004764. [DOI] [PMC free article] [PubMed] [Google Scholar]
- HODGKIN A. L., HUXLEY A. F. Currents carried by sodium and potassium ions through the membrane of the giant axon of Loligo. J Physiol. 1952 Apr;116(4):449–472. doi: 10.1113/jphysiol.1952.sp004717. [DOI] [PMC free article] [PubMed] [Google Scholar]
- HODGKIN A. L., KATZ B. The effect of sodium ions on the electrical activity of giant axon of the squid. J Physiol. 1949 Mar 1;108(1):37–77. doi: 10.1113/jphysiol.1949.sp004310. [DOI] [PMC free article] [PubMed] [Google Scholar]
- HODGKIN A. L., KEYNES R. D. The potassium permeability of a giant nerve fibre. J Physiol. 1955 Apr 28;128(1):61–88. doi: 10.1113/jphysiol.1955.sp005291. [DOI] [PMC free article] [PubMed] [Google Scholar]
- Hille B. Ionic selectivity, saturation, and block in sodium channels. A four-barrier model. J Gen Physiol. 1975 Nov;66(5):535–560. doi: 10.1085/jgp.66.5.535. [DOI] [PMC free article] [PubMed] [Google Scholar]
- Hille B., Schwarz W. Potassium channels as multi-ion single-file pores. J Gen Physiol. 1978 Oct;72(4):409–442. doi: 10.1085/jgp.72.4.409. [DOI] [PMC free article] [PubMed] [Google Scholar]
- Horowicz P., Gage P. W., Eisenberg R. S. The role of the electrochemical gradient in determining potassium fluxes in frog striated muscle. J Gen Physiol. 1968 May 1;51(5):193–203. [PMC free article] [PubMed] [Google Scholar]
- Kohler H. H., Heckmann K. Unidirectional fluxes in saturated single-file pores of biological and artificial membranes. I. Pores containing no more than one vacancy. J Theor Biol. 1979 Aug 7;79(3):381–401. doi: 10.1016/0022-5193(79)90354-0. [DOI] [PubMed] [Google Scholar]
- Kukita F. Removal of periaxonal potassium accumulation in a squid giant axon by outward osmotic water flow. J Physiol. 1988 May;399:647–656. doi: 10.1113/jphysiol.1988.sp017101. [DOI] [PMC free article] [PubMed] [Google Scholar]
- Latorre R., Miller C. Conduction and selectivity in potassium channels. J Membr Biol. 1983;71(1-2):11–30. doi: 10.1007/BF01870671. [DOI] [PubMed] [Google Scholar]
- Neyton J., Miller C. Discrete Ba2+ block as a probe of ion occupancy and pore structure in the high-conductance Ca2+ -activated K+ channel. J Gen Physiol. 1988 Nov;92(5):569–586. doi: 10.1085/jgp.92.5.569. [DOI] [PMC free article] [PubMed] [Google Scholar]
- Schumaker M. F., MacKinnon R. A simple model for multi-ion permeation. Single-vacancy conduction in a simple pore model. Biophys J. 1990 Oct;58(4):975–984. doi: 10.1016/S0006-3495(90)82442-5. [DOI] [PMC free article] [PubMed] [Google Scholar]
- Siegelbaum S. A., Camardo J. S., Kandel E. R. Serotonin and cyclic AMP close single K+ channels in Aplysia sensory neurones. Nature. 1982 Sep 30;299(5882):413–417. doi: 10.1038/299413a0. [DOI] [PubMed] [Google Scholar]
- Swenson R. P., Jr, Armstrong C. M. K+ channels close more slowly in the presence of external K+ and Rb+. Nature. 1981 Jun 4;291(5814):427–429. doi: 10.1038/291427a0. [DOI] [PubMed] [Google Scholar]
- Vestergaard-Bogind B., Stampe P., Christophersen P. Single-file diffusion through the Ca2+-activated K+ channel of human red cells. J Membr Biol. 1985;88(1):67–75. doi: 10.1007/BF01871214. [DOI] [PubMed] [Google Scholar]
- Wagoner P. K., Oxford G. S. Cation permeation through the voltage-dependent potassium channel in the squid axon. Characteristics and mechanisms. J Gen Physiol. 1987 Aug;90(2):261–290. doi: 10.1085/jgp.90.2.261. [DOI] [PMC free article] [PubMed] [Google Scholar]