Abstract
Previous work from this laboratory demonstrated that the environment-sensitive lysolipid N-(7-nitrobenz-2-oxa-1,3-diazol-4-yl)- monomyristoylphosphatidylethanolamine (N-NBD-MPE), at concentrations below its critical micelle concentration (CMCN-NBD-MPE = 4 microM), reached maximum fluorescence yield upon the addition of taurodeoxycholate (TDC) at concentrations well below its CMC (CMCTDC = 2.5 mM). These data indicated the formation of micellar aggregates of the two amphiphiles at concentrations below both of their CMCs. In the present study, fluorescence lifetime and differential polarization measurements were made to determine the size of these aggregates. In the absence of TDC and at 0.5 mM TDC a single lifetime (tau) and rotational correlation time (phi) were measured for N-NBD-MPE at the submicellar concentration of 2 microM, indicating a lack of interaction between the two molecules at this concentration. Above 0.5 mM TDC, two discrete lifetimes were resolved. Based on these lifetimes, two distinct rotational correlation times were established through polarization measurements. The shorter phi(0.19-0.73 ns) was ascribed to local probe motions, whereas the longer phi was in a time range expected for global rotation of aggregates the size of simple bile salt micelles (3-6.5 ns). From the longer phi, molecular volume and hydrodynamic radii were calculated, ranging from approximately 15 A at 1 mM to approximately 18 A at 5 mM TDC. These data support the conclusion that monomeric lysolipids in solution seed the aggregation of numerous TDC molecules (aggregation number = 16 at 1 mM TDC) to form a TDC micelle with a lysolipid core at concentrations below which they both self-aggregate.
Full text
PDF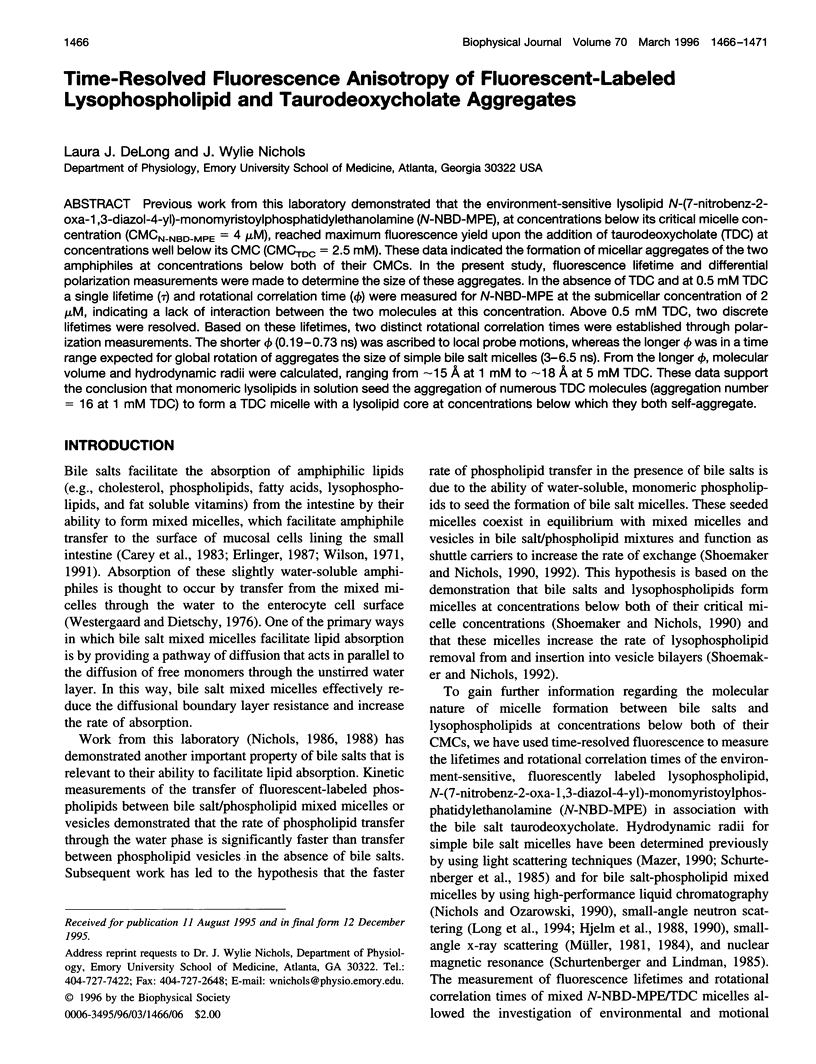
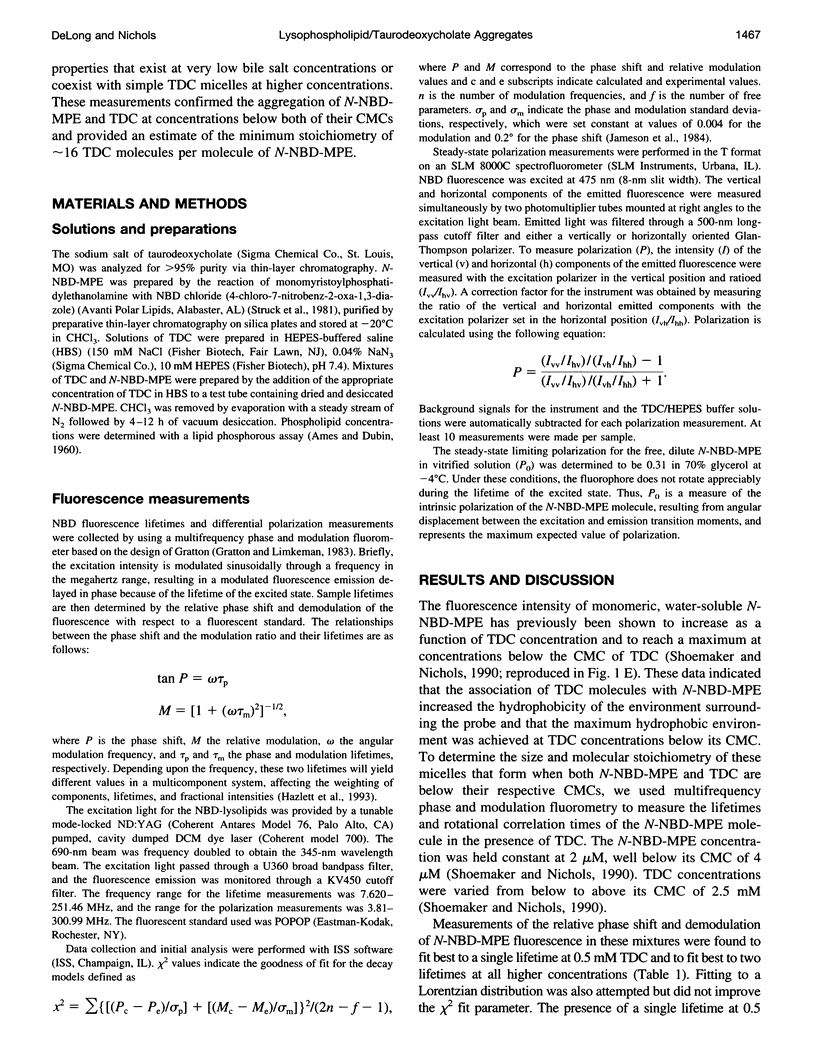
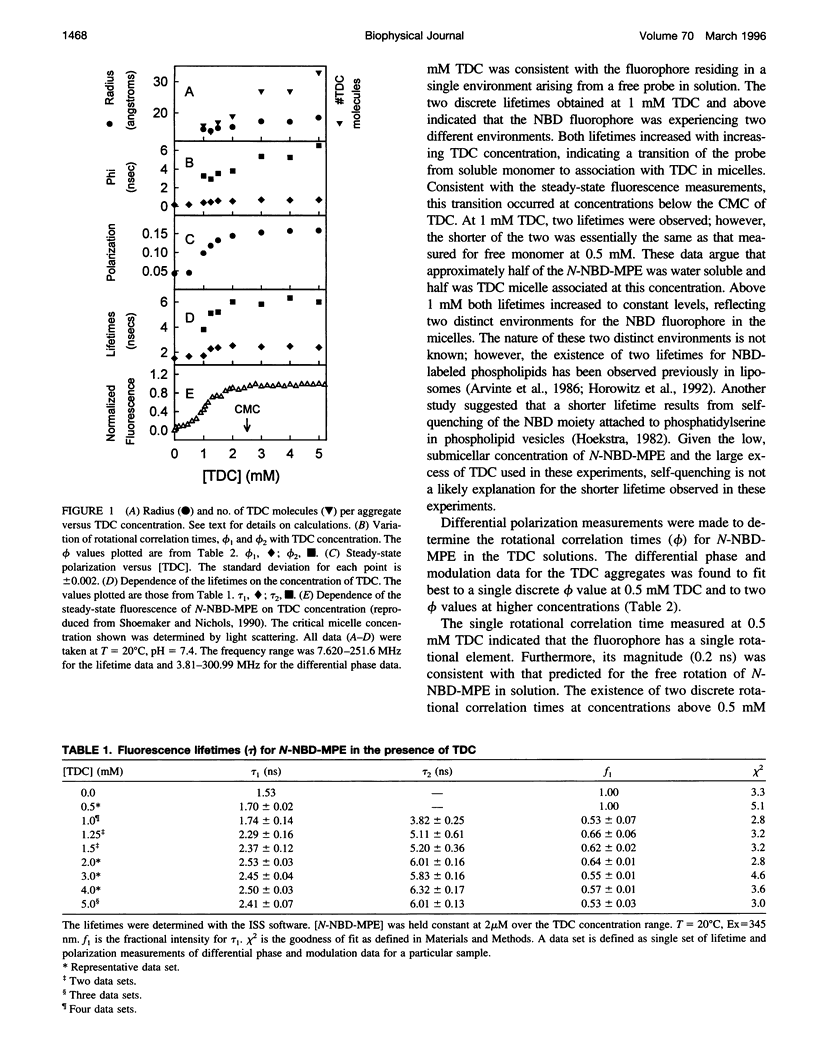
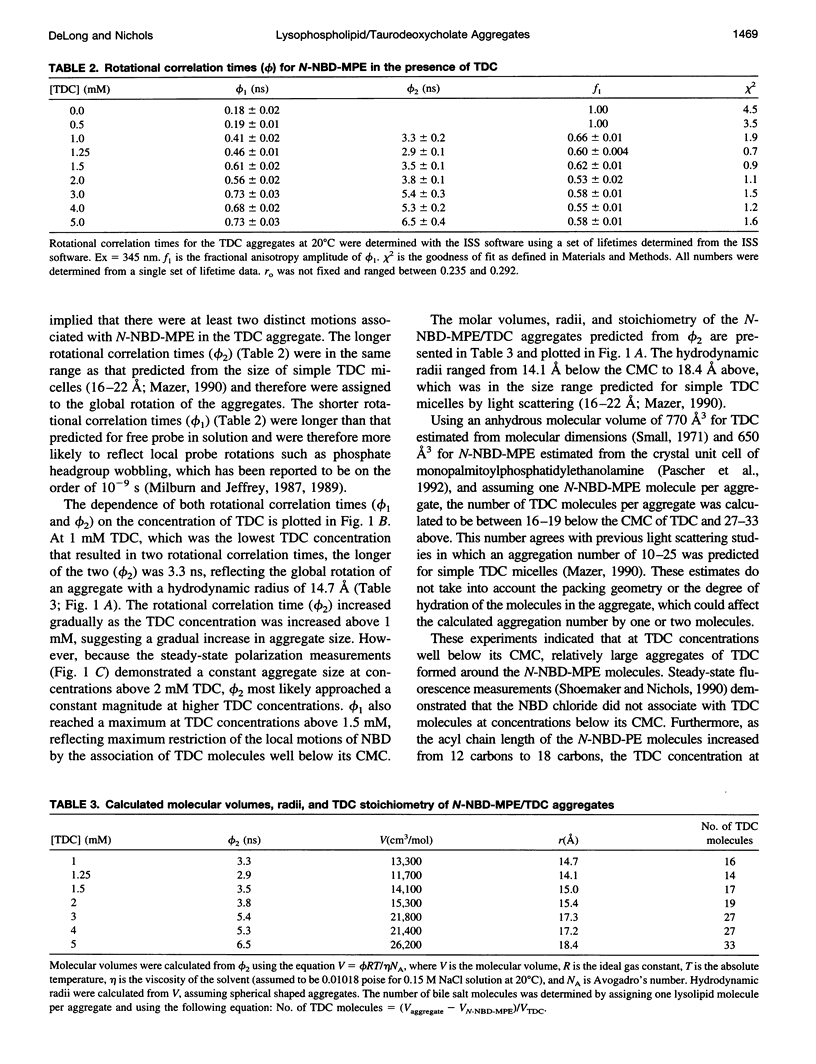
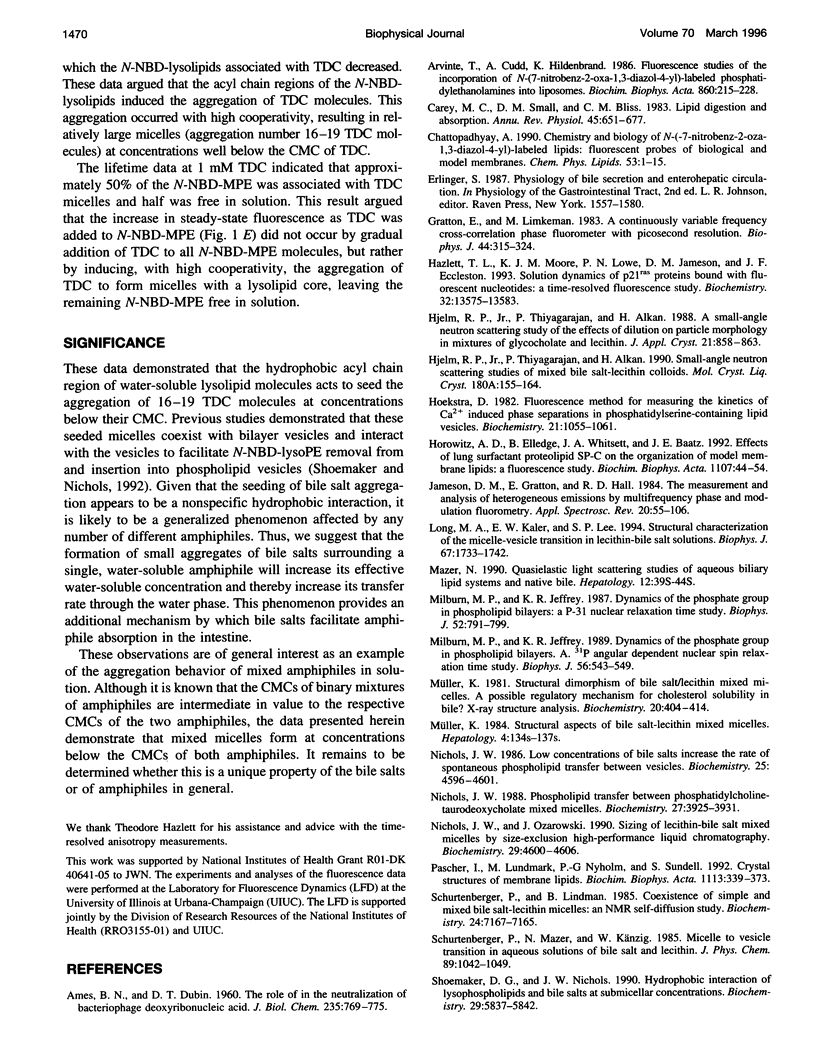
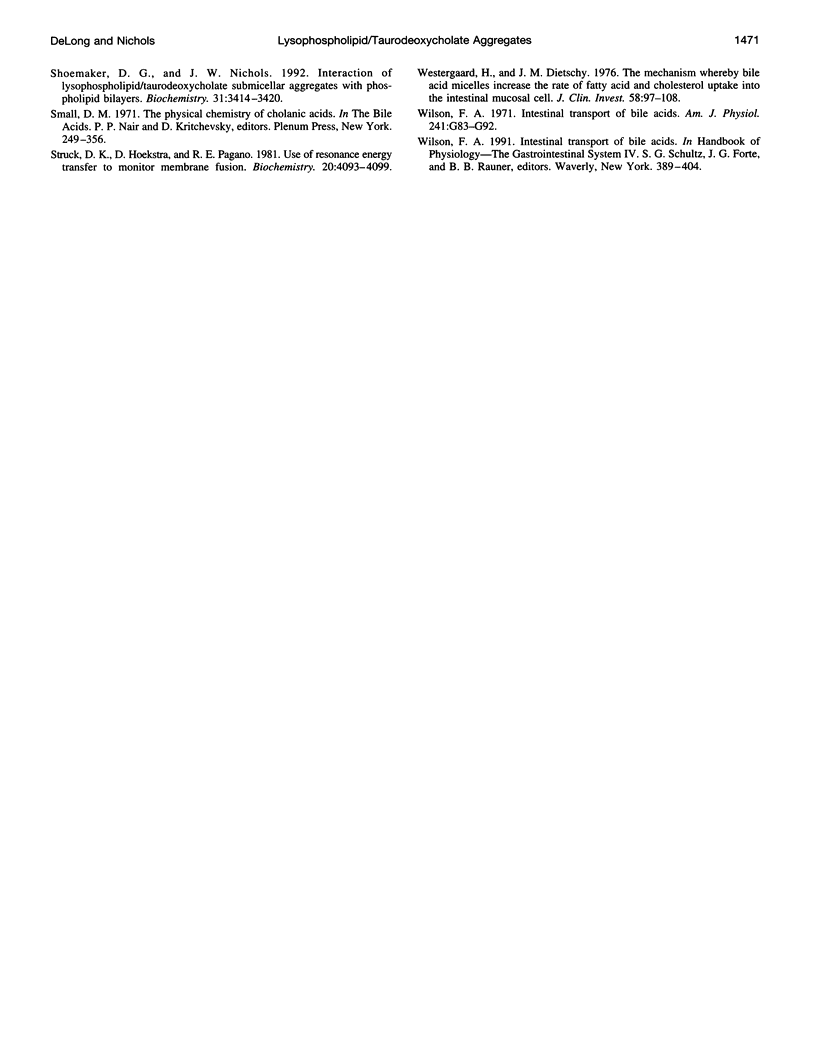
Selected References
These references are in PubMed. This may not be the complete list of references from this article.
- AMES B. N., DUBIN D. T. The role of polyamines in the neutralization of bacteriophage deoxyribonucleic acid. J Biol Chem. 1960 Mar;235:769–775. [PubMed] [Google Scholar]
- Carey M. C., Small D. M., Bliss C. M. Lipid digestion and absorption. Annu Rev Physiol. 1983;45:651–677. doi: 10.1146/annurev.ph.45.030183.003251. [DOI] [PubMed] [Google Scholar]
- Chattopadhyay A. Chemistry and biology of N-(7-nitrobenz-2-oxa-1,3-diazol-4-yl)-labeled lipids: fluorescent probes of biological and model membranes. Chem Phys Lipids. 1990 Mar;53(1):1–15. doi: 10.1016/0009-3084(90)90128-e. [DOI] [PubMed] [Google Scholar]
- Gratton E., Limkeman M. A continuously variable frequency cross-correlation phase fluorometer with picosecond resolution. Biophys J. 1983 Dec;44(3):315–324. doi: 10.1016/S0006-3495(83)84305-7. [DOI] [PMC free article] [PubMed] [Google Scholar]
- Hazlett T. L., Moore K. J., Lowe P. N., Jameson D. M., Eccleston J. F. Solution dynamics of p21ras proteins bound with fluorescent nucleotides: a time-resolved fluorescence study. Biochemistry. 1993 Dec 14;32(49):13575–13583. doi: 10.1021/bi00212a025. [DOI] [PubMed] [Google Scholar]
- Hoekstra D. Fluorescence method for measuring the kinetics of Ca2+-induced phase separations in phosphatidylserine-containing lipid vesicles. Biochemistry. 1982 Mar 2;21(5):1055–1061. doi: 10.1021/bi00534a036. [DOI] [PubMed] [Google Scholar]
- Horowitz A. D., Elledge B., Whitsett J. A., Baatz J. E. Effects of lung surfactant proteolipid SP-C on the organization of model membrane lipids: a fluorescence study. Biochim Biophys Acta. 1992 Jun 11;1107(1):44–54. doi: 10.1016/0005-2736(92)90327-i. [DOI] [PubMed] [Google Scholar]
- Long M. A., Kaler E. W., Lee S. P. Structural characterization of the micelle-vesicle transition in lecithin-bile salt solutions. Biophys J. 1994 Oct;67(4):1733–1742. doi: 10.1016/S0006-3495(94)80647-2. [DOI] [PMC free article] [PubMed] [Google Scholar]
- Mazer N. A. Quasielastic light scattering studies of aqueous biliary lipid systems and native bile. Hepatology. 1990 Sep;12(3 Pt 2):39S–44S. [PubMed] [Google Scholar]
- Milburn M. P., Jeffrey K. R. Dynamics of the phosphate group in phospholipid bilayers. A 31P angular dependent nuclear spin relaxation time study. Biophys J. 1989 Sep;56(3):543–549. doi: 10.1016/S0006-3495(89)82701-8. [DOI] [PMC free article] [PubMed] [Google Scholar]
- Milburn M. P., Jeffrey K. R. Dynamics of the phosphate group in phospholipid bilayers. A 31P nuclear relaxation time study. Biophys J. 1987 Nov;52(5):791–799. doi: 10.1016/S0006-3495(87)83273-3. [DOI] [PMC free article] [PubMed] [Google Scholar]
- Müller K. Structural aspects of bile salt-lecithin mixed micelles. Hepatology. 1984 Sep-Oct;4(5 Suppl):134S–137S. doi: 10.1002/hep.1840040823. [DOI] [PubMed] [Google Scholar]
- Müller K. Structural dimorphism of bile salt/lecithin mixed micelles. A possible regulatory mechanism for cholesterol solubility in bile? X-ray structure analysis. Biochemistry. 1981 Jan 20;20(2):404–414. doi: 10.1021/bi00505a028. [DOI] [PubMed] [Google Scholar]
- Nichols J. W. Low concentrations of bile salts increase the rate of spontaneous phospholipid transfer between vesicles. Biochemistry. 1986 Aug 12;25(16):4596–4601. doi: 10.1021/bi00364a021. [DOI] [PubMed] [Google Scholar]
- Nichols J. W., Ozarowski J. Sizing of lecithin-bile salt mixed micelles by size-exclusion high-performance liquid chromatography. Biochemistry. 1990 May 15;29(19):4600–4606. doi: 10.1021/bi00471a014. [DOI] [PubMed] [Google Scholar]
- Nichols J. W. Phospholipid transfer between phosphatidylcholine-taurocholate mixed micelles. Biochemistry. 1988 May 31;27(11):3925–3931. doi: 10.1021/bi00411a006. [DOI] [PubMed] [Google Scholar]
- Pascher I., Lundmark M., Nyholm P. G., Sundell S. Crystal structures of membrane lipids. Biochim Biophys Acta. 1992 Dec 11;1113(3-4):339–373. doi: 10.1016/0304-4157(92)90006-v. [DOI] [PubMed] [Google Scholar]
- Schurtenberger P., Lindman B. Coexistence of simple and mixed bile salt-lecithin micelles: an NMR self-diffusion study. Biochemistry. 1985 Dec 3;24(25):7161–7165. doi: 10.1021/bi00346a021. [DOI] [PubMed] [Google Scholar]
- Shoemaker D. G., Nichols J. W. Hydrophobic interaction of lysophospholipids and bile salts at submicellar concentrations. Biochemistry. 1990 Jun 19;29(24):5837–5842. doi: 10.1021/bi00476a027. [DOI] [PubMed] [Google Scholar]
- Shoemaker D. G., Nichols J. W. Interaction of lysophospholipid/taurodeoxycholate submicellar aggregates with phospholipid bilayers. Biochemistry. 1992 Apr 7;31(13):3414–3420. doi: 10.1021/bi00128a016. [DOI] [PubMed] [Google Scholar]
- Struck D. K., Hoekstra D., Pagano R. E. Use of resonance energy transfer to monitor membrane fusion. Biochemistry. 1981 Jul 7;20(14):4093–4099. doi: 10.1021/bi00517a023. [DOI] [PubMed] [Google Scholar]
- Westergaard H., Dietschy J. M. The mechanism whereby bile acid micelles increase the rate of fatty acid and cholesterol uptake into the intestinal mucosal cell. J Clin Invest. 1976 Jul;58(1):97–108. doi: 10.1172/JCI108465. [DOI] [PMC free article] [PubMed] [Google Scholar]
- Wilson F. A. Intestinal transport of bile acids. Am J Physiol. 1981 Aug;241(2):G83–G92. doi: 10.1152/ajpgi.1981.241.2.G83. [DOI] [PubMed] [Google Scholar]