Abstract
1. Electrical coupling of Rohon-Beard neurones in the spinal cord of Xenopus laevis has been studied from the time of closure of the neural tube (stage 20, 22h after fertilization of the egg) to the free swimming tadpole (stage 49, 12d old). Pairs of cells were examined by impaling both simultaneously with single micro-electrodes having current-passing and voltage-recording capabilities.
2. At the earliest time studied (stage 20), before Ca2+ action potentials are detected, cells are electrically coupled. Coupling coefficients as high as 0·6 can be recorded. Tests with small hyperpolarizing and depolarizing current pulses demonstrate that the coupling does not show rectification.
3. The coupling is voltage-dependent: depolarization or hyperpolarization of one cell with respect to another, above a threshold, causes relative uncoupling of the cells. The coupling coefficient falls to ∼10% of its initial value when the difference in potential between the two cell bodies is ∼75 mV. Cells usually become recoupled at the termination of the current pulse. Other, unidentified cells in the spinal cords of the same embryos show coupling that is not voltage-dependent.
4. Voltage-dependent uncoupling and recoupling persist when cells are depolarized by high K+, and in the presence of 30 mM-Co2+, suggesting that chemical synapses are not involved. They are also unaffected by addition of Rb+, Cs+ or TEA+ to the extracellular solution, elevated levels of Ca2+, or replacement of Na+ with Tris or Cl- with isethionate, suggesting that conductance changes in the surface membrane, such as anomalous rectification, are not responsible.
5. Lowering the intracellular pH with CO2-HCO3- buffered saline does not abolish electrical coupling but appears to eliminate its voltage dependence.
6. Slightly later (e.g. stage 21), cells that do not yet produce Ca2+ action potentials while coupled will do so when their input resistance is increased by uncoupling them from their neighbours.
7. Later still (e.g. stage 23), cells make Ca2+ action potentials while coupled, and an action potential in one cell can trigger an action potential in other cells to which it is coupled. Ca2+ action potentials that do not bring other coupled cells to threshold for impulse initiation can transiently reduce the strength of coupling. Repetitive firing of these Ca2+ action potentials at a low frequency does not cause permanent uncoupling of the cells.
8. Rohon-Beard neurones become electrically uncoupled about stage 25 (early tail-bud, 28h old). Coupling disappears around the time of appearance of the Na+ component of the action potential, although coupling that is voltage-dependent or independent can still be seen between other, unidentified cells. No electrical coupling of Rohon-Beard cells was detected at later stages of development.
Full text
PDF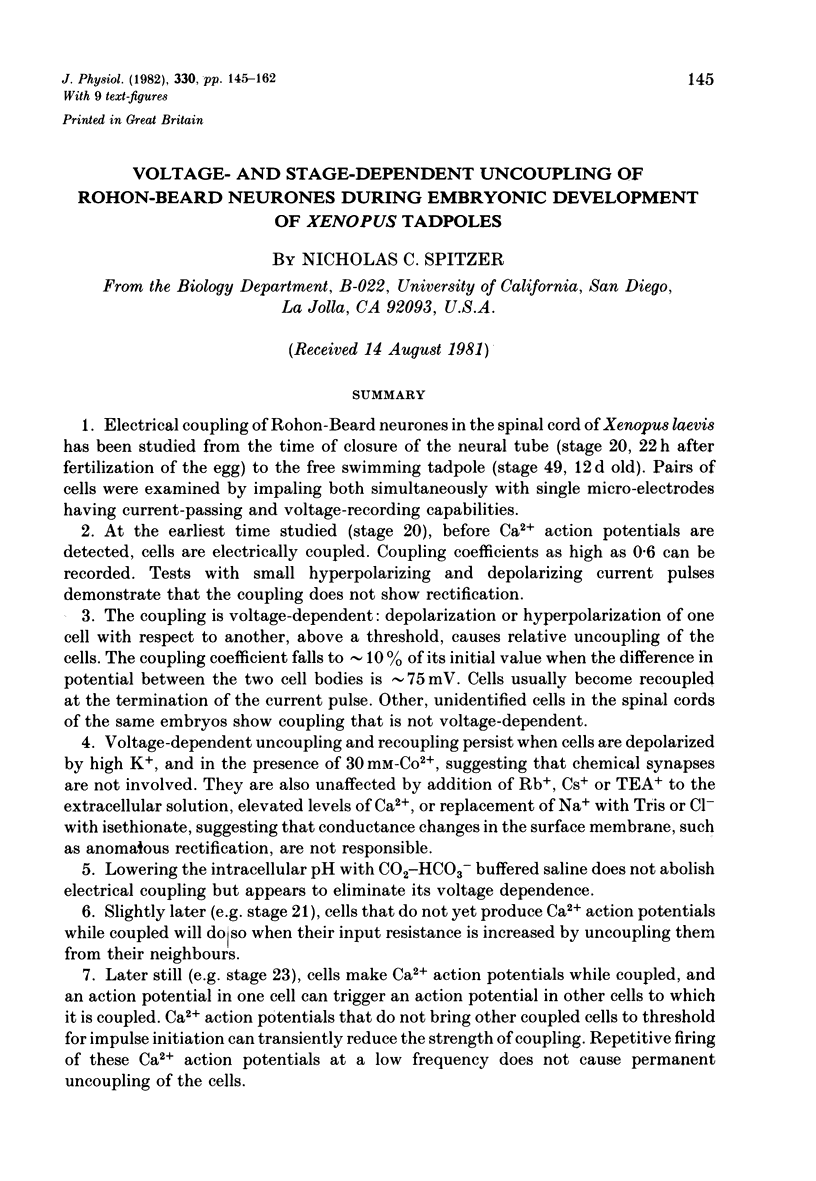
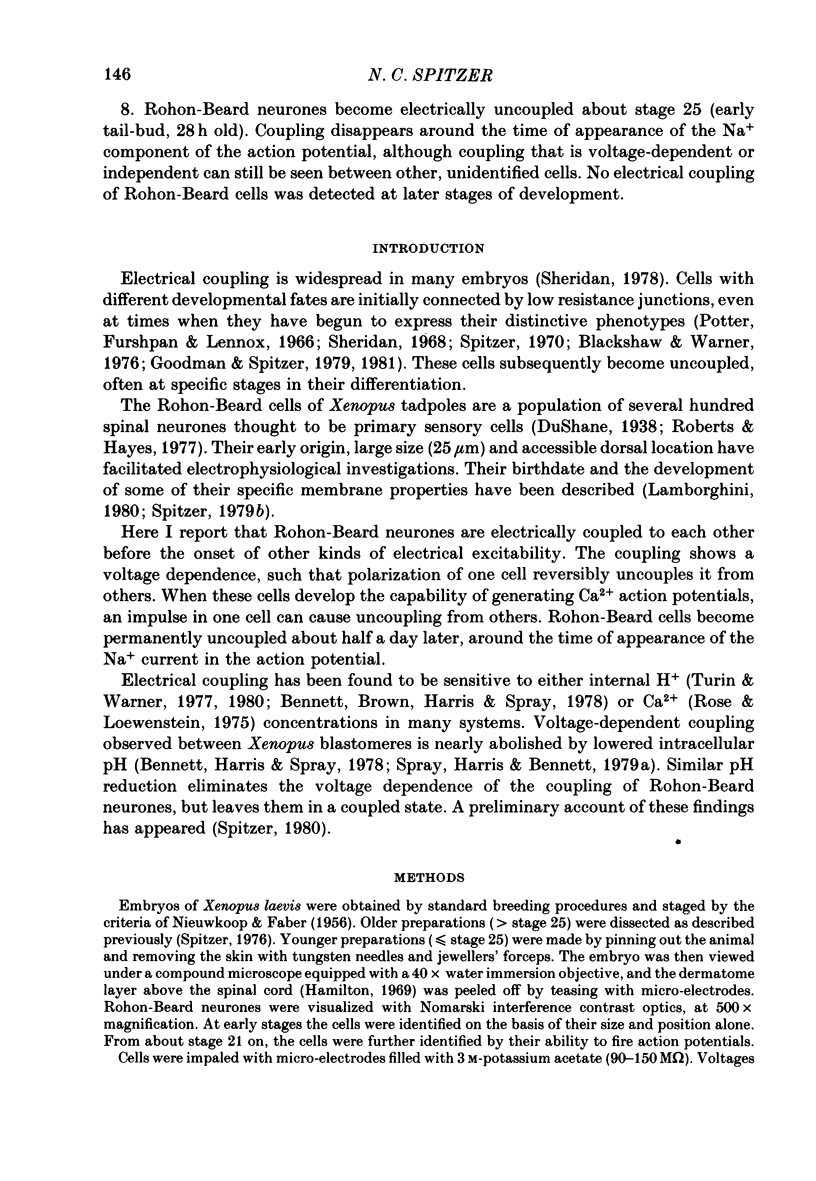
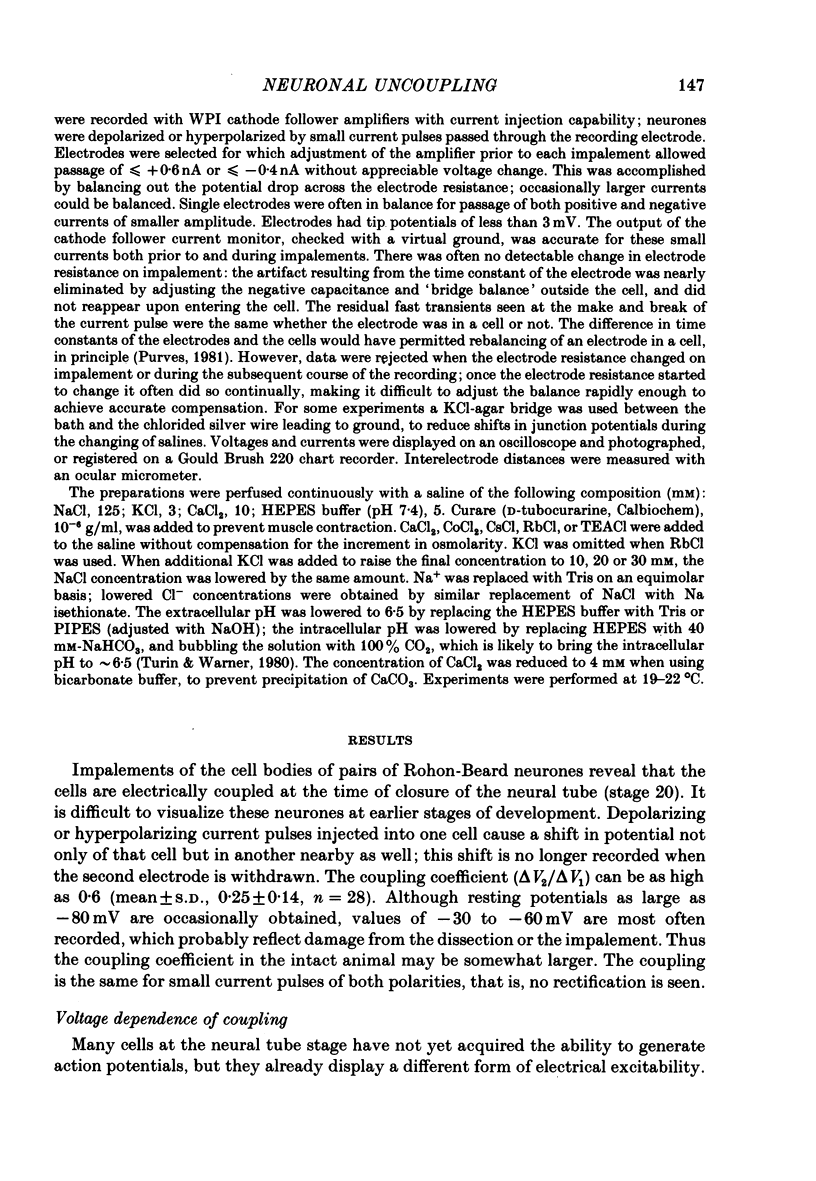
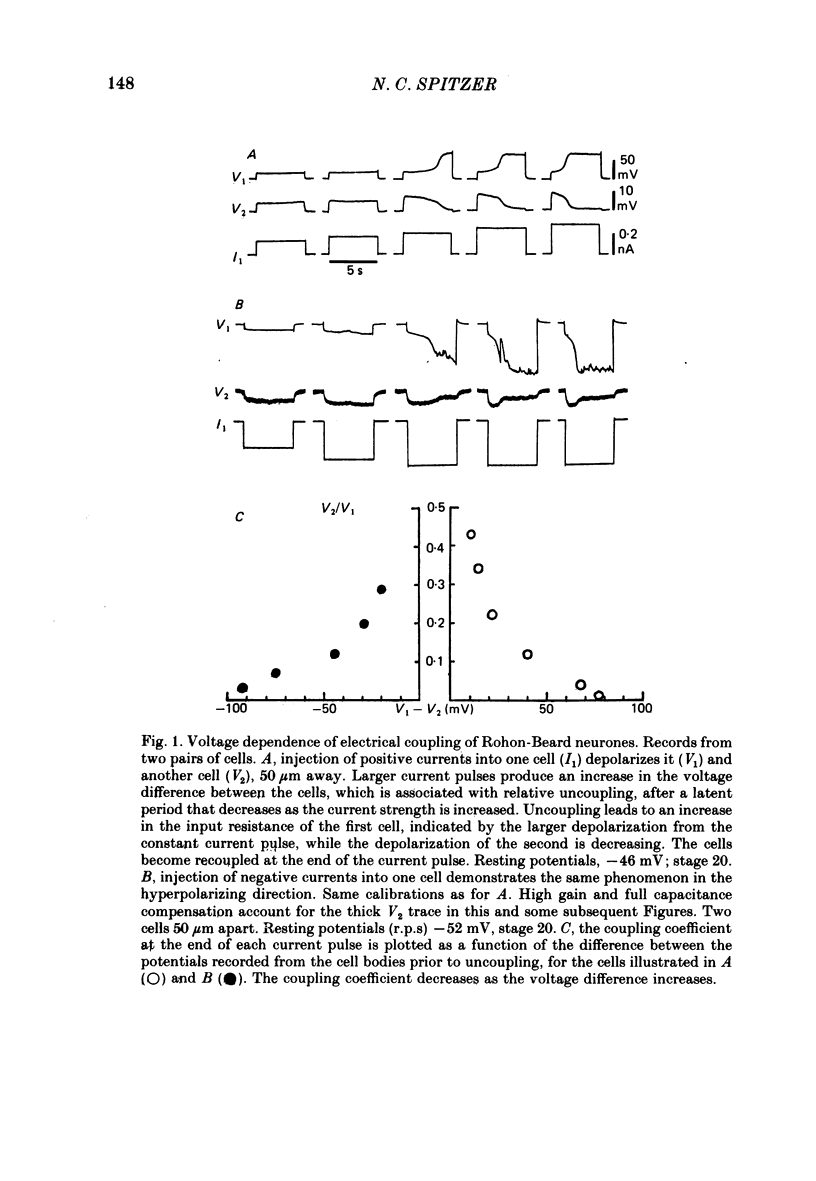
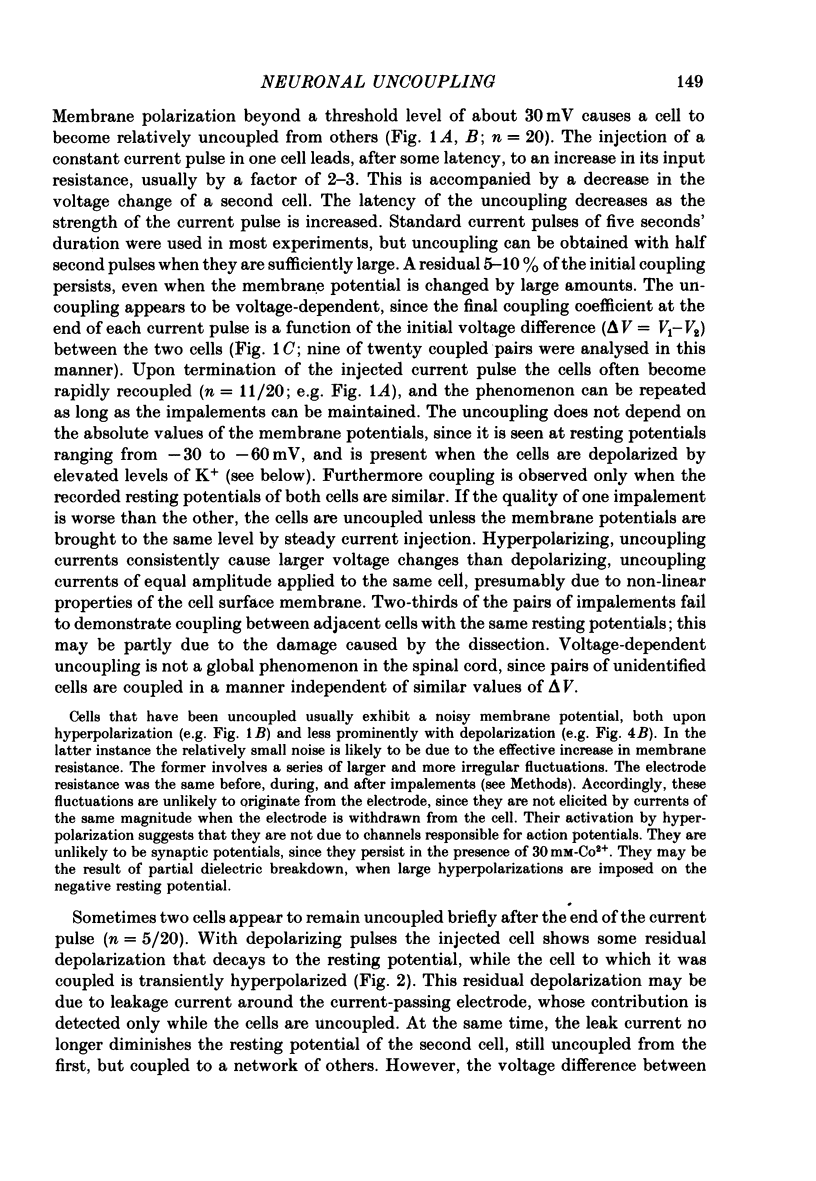
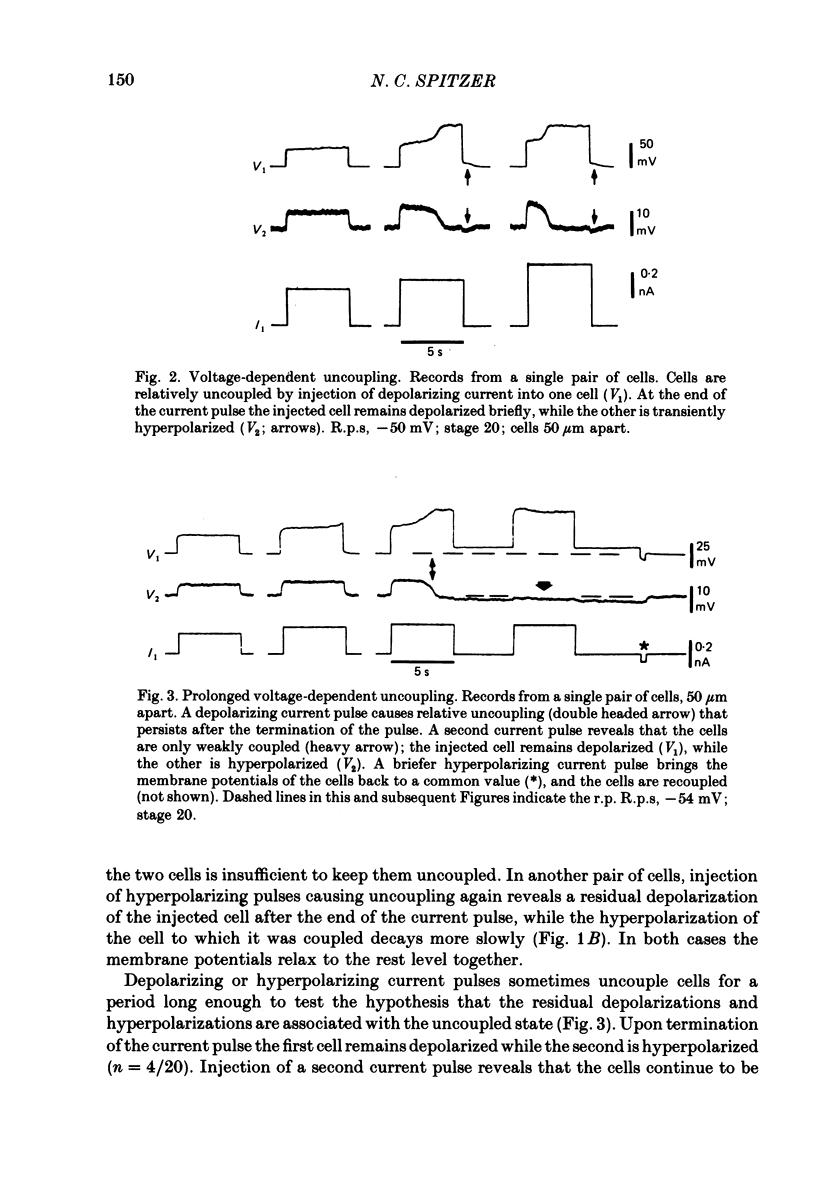
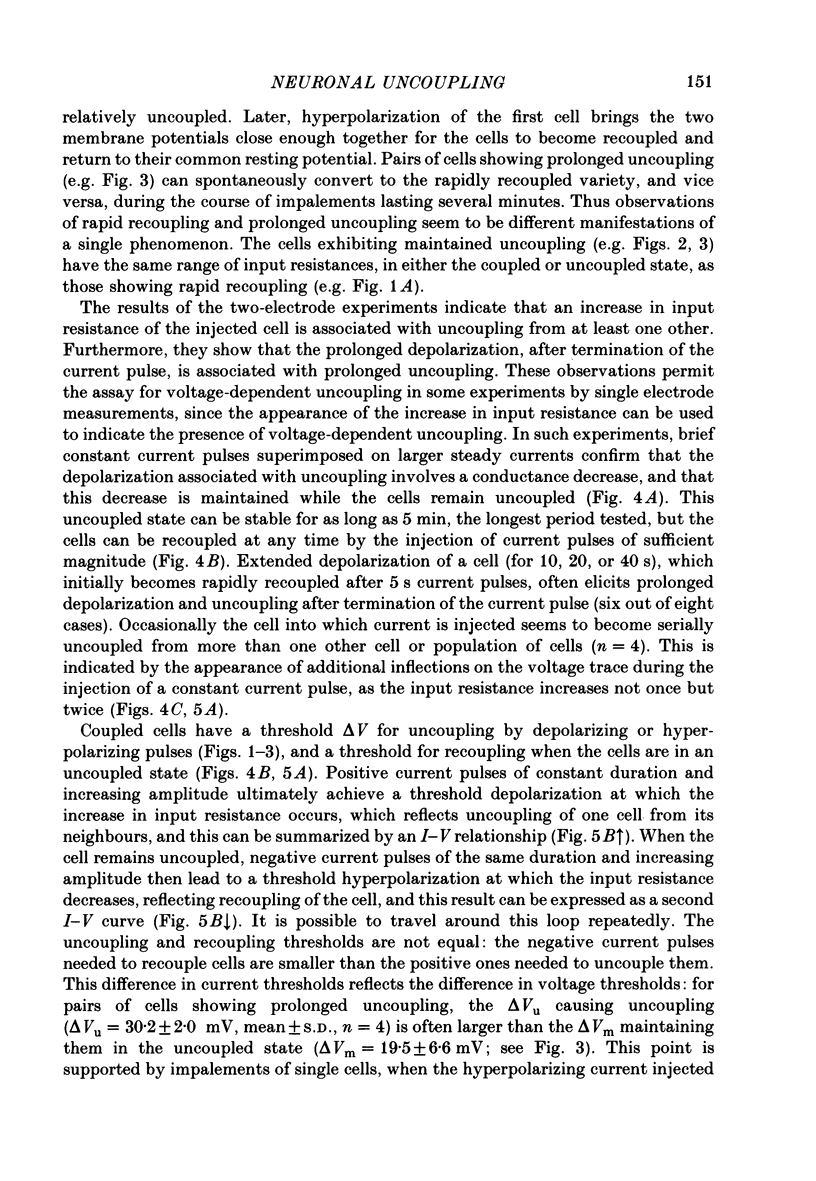
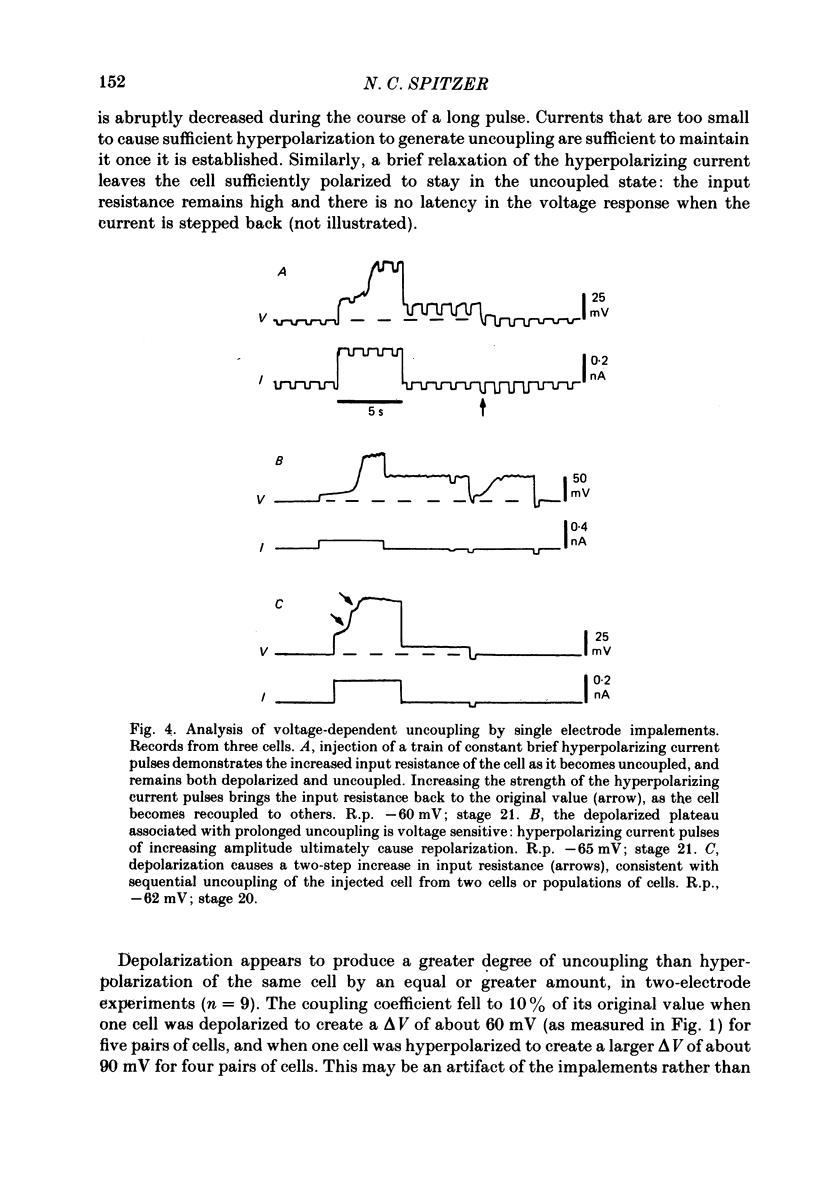
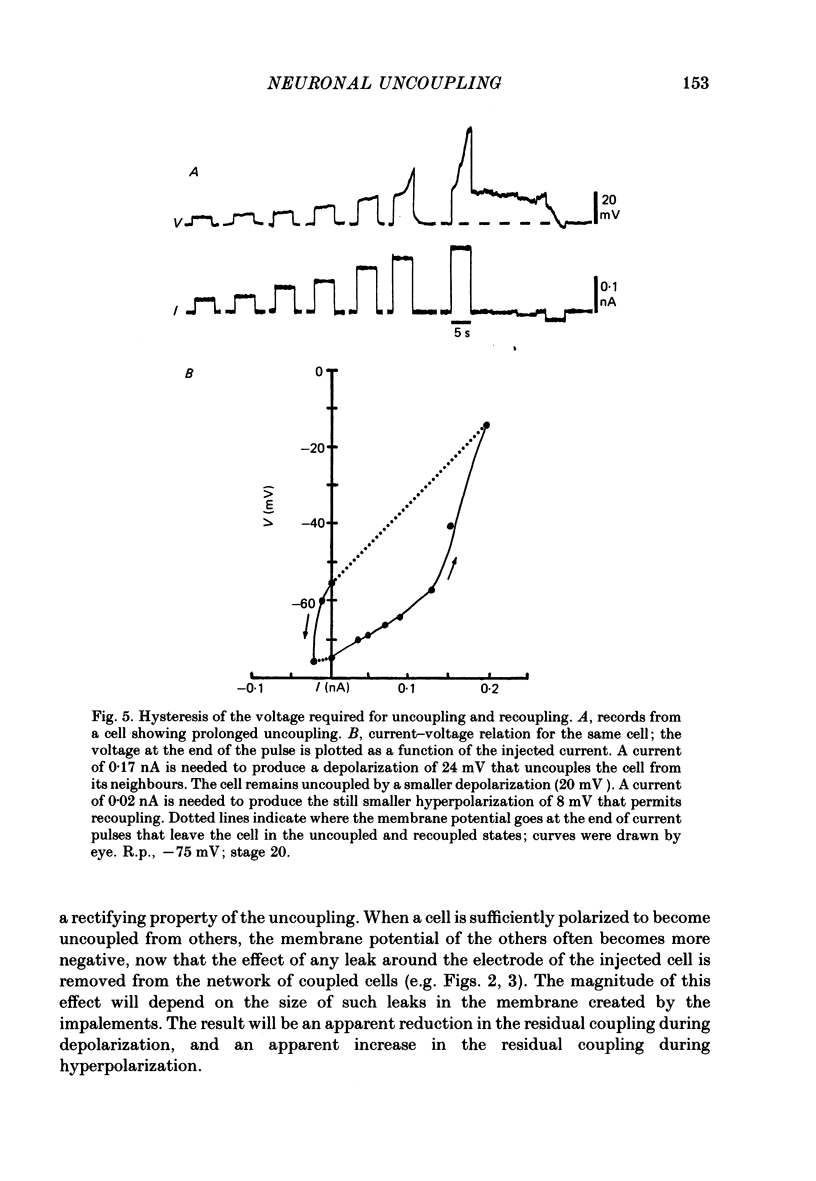
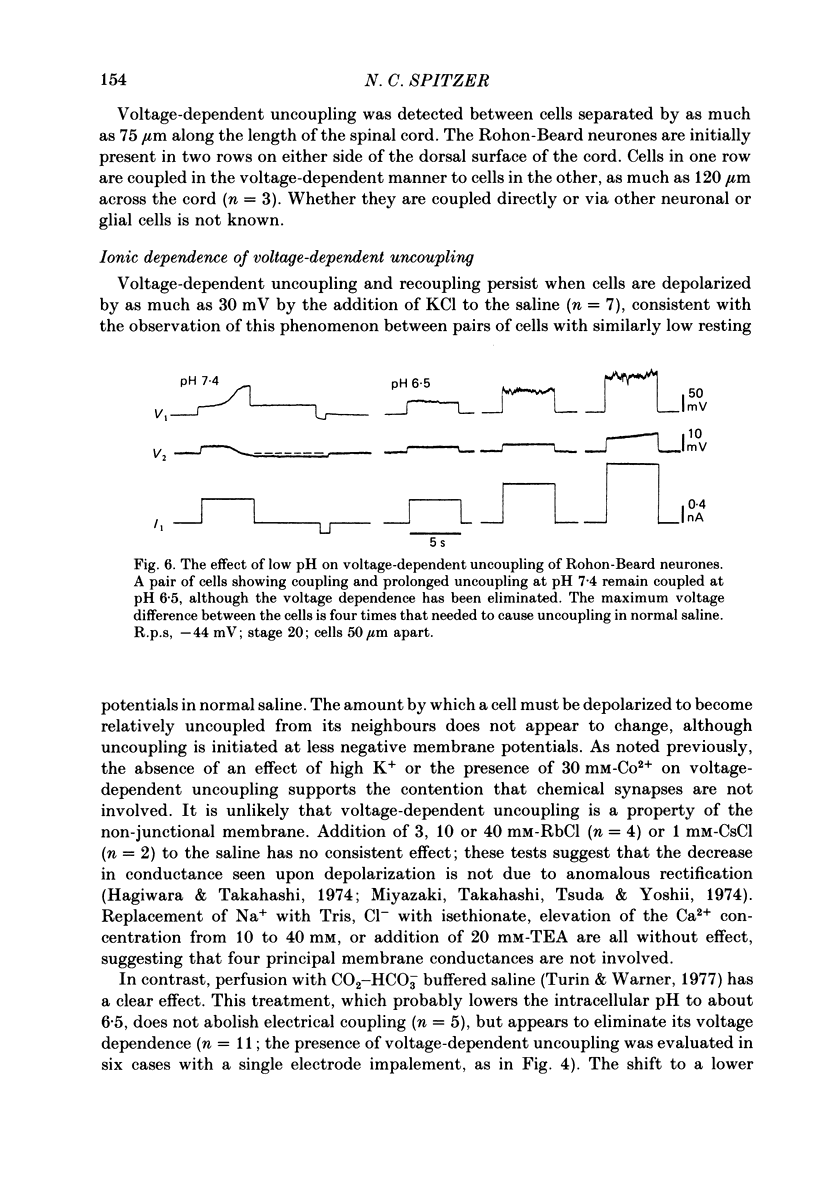
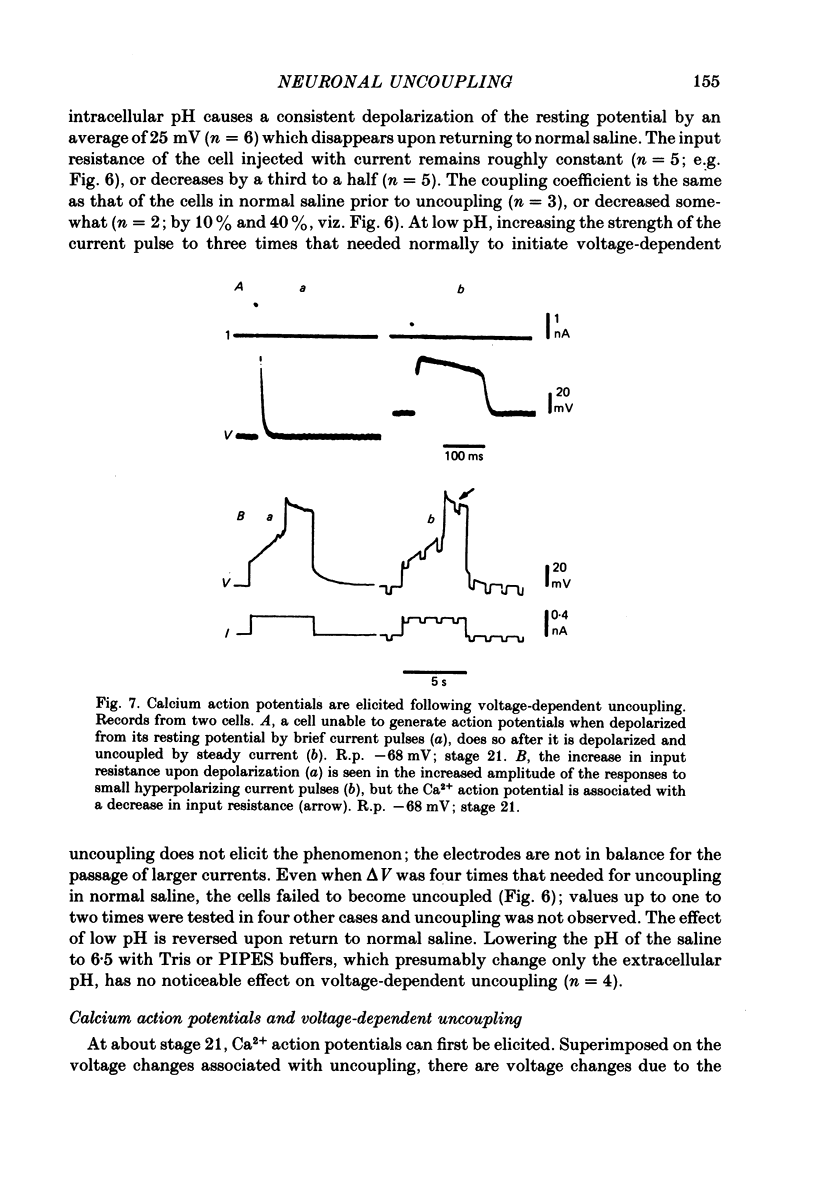
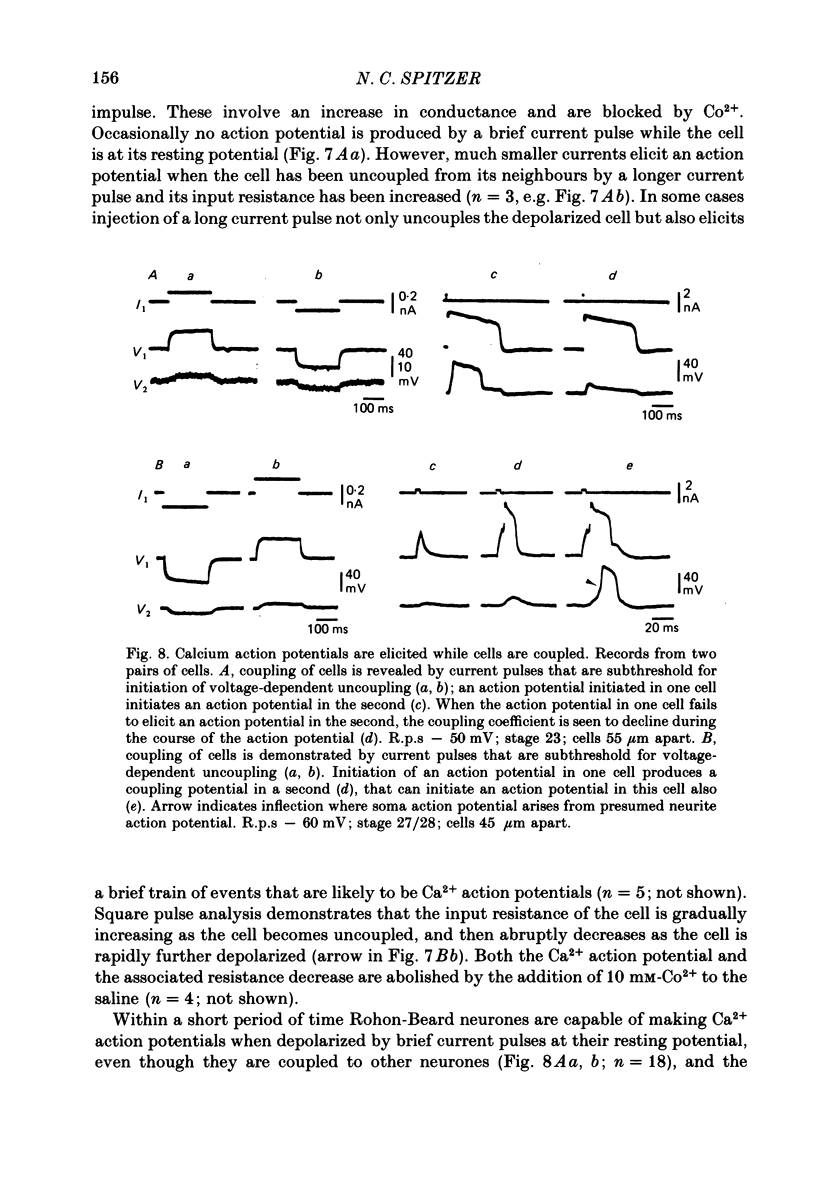
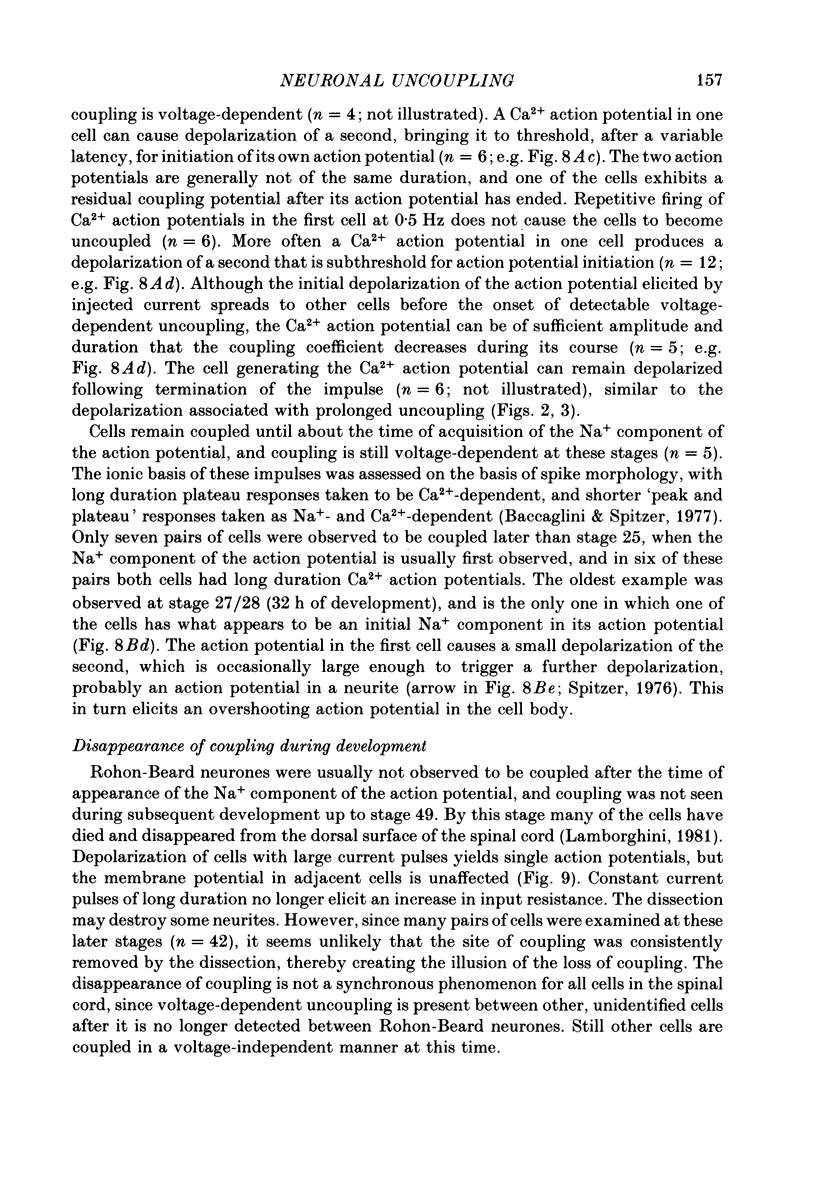
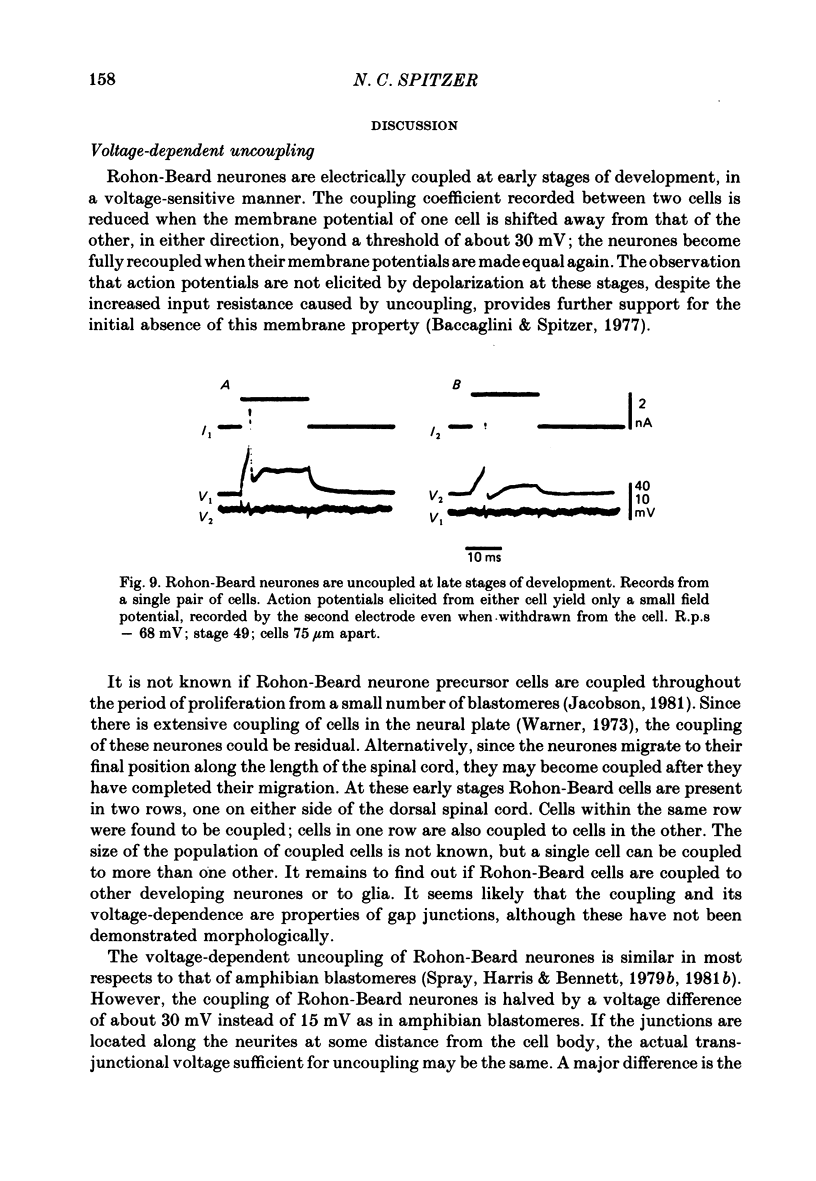
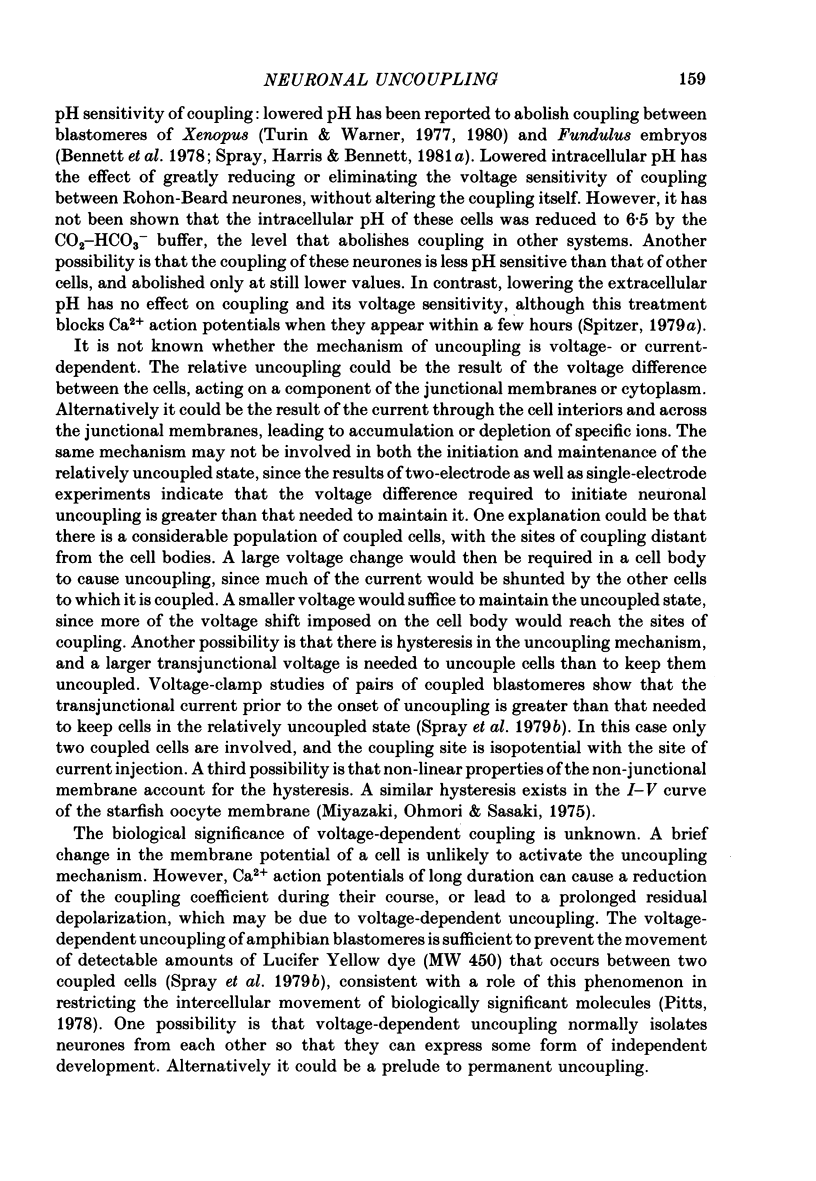
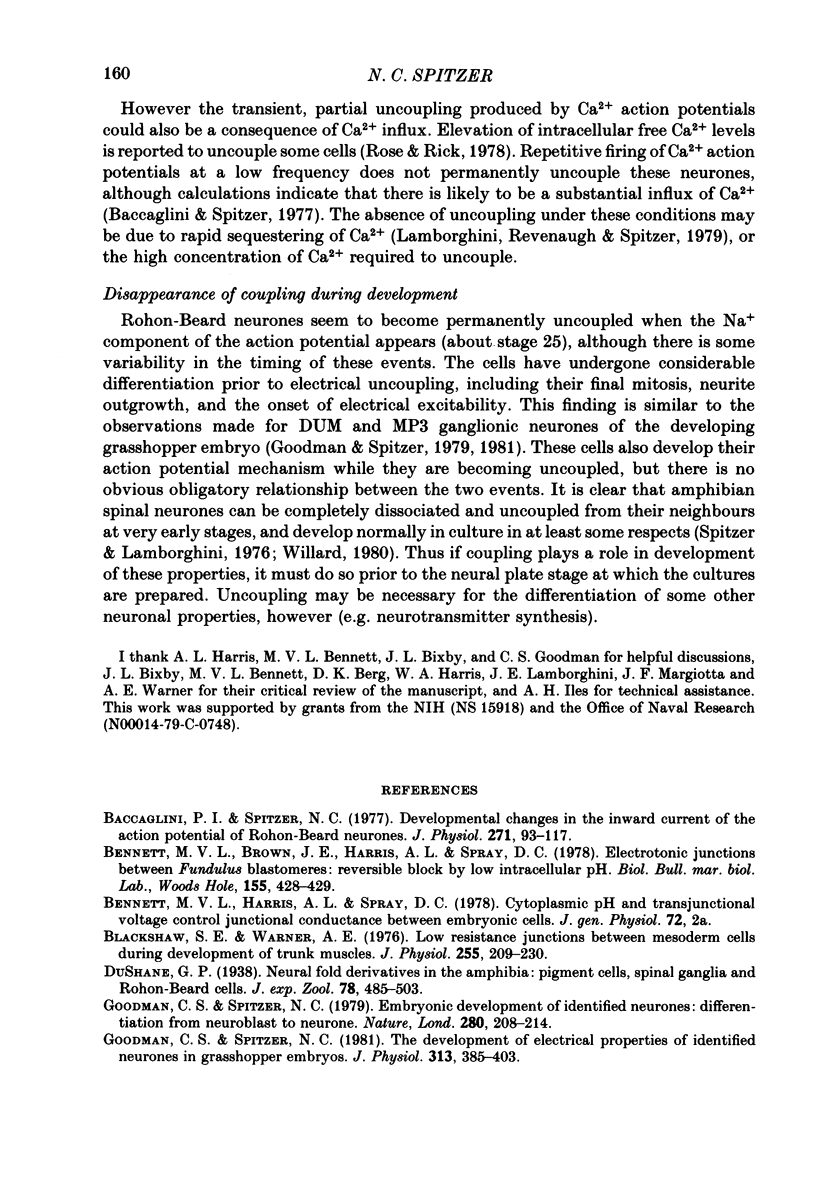
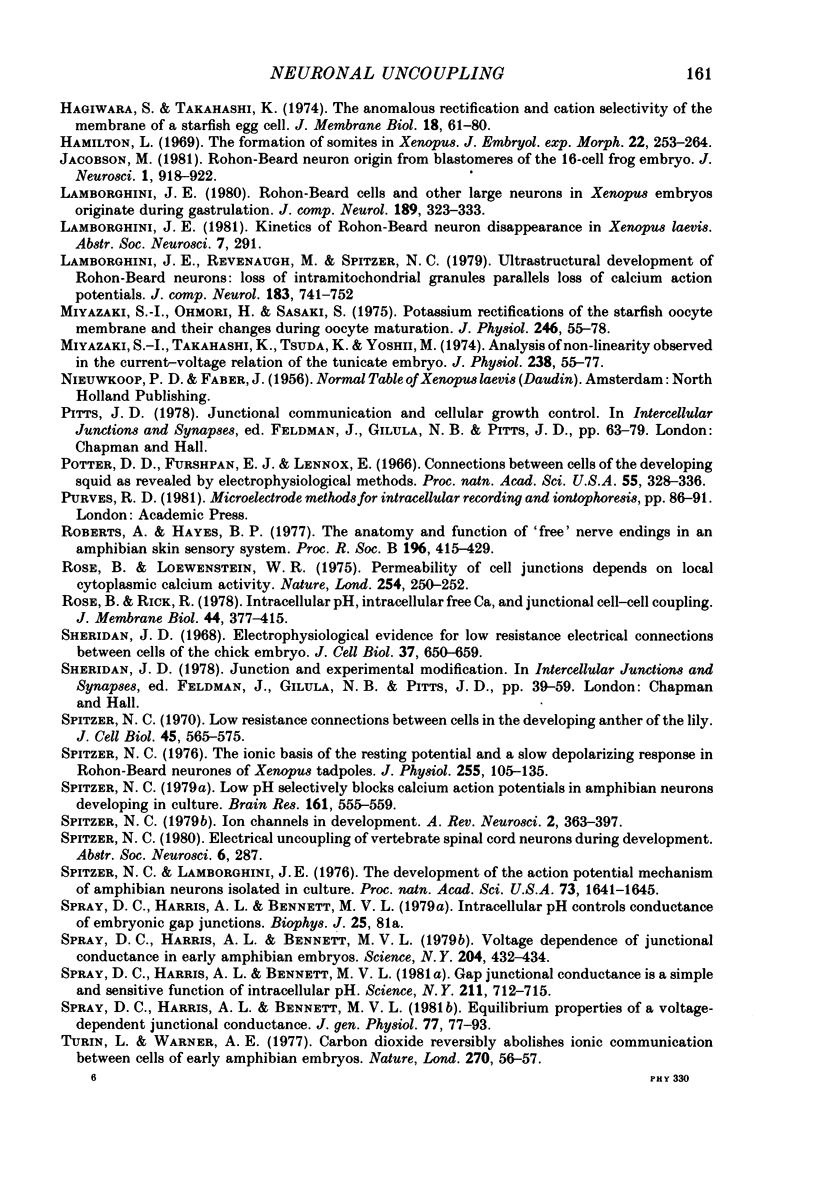
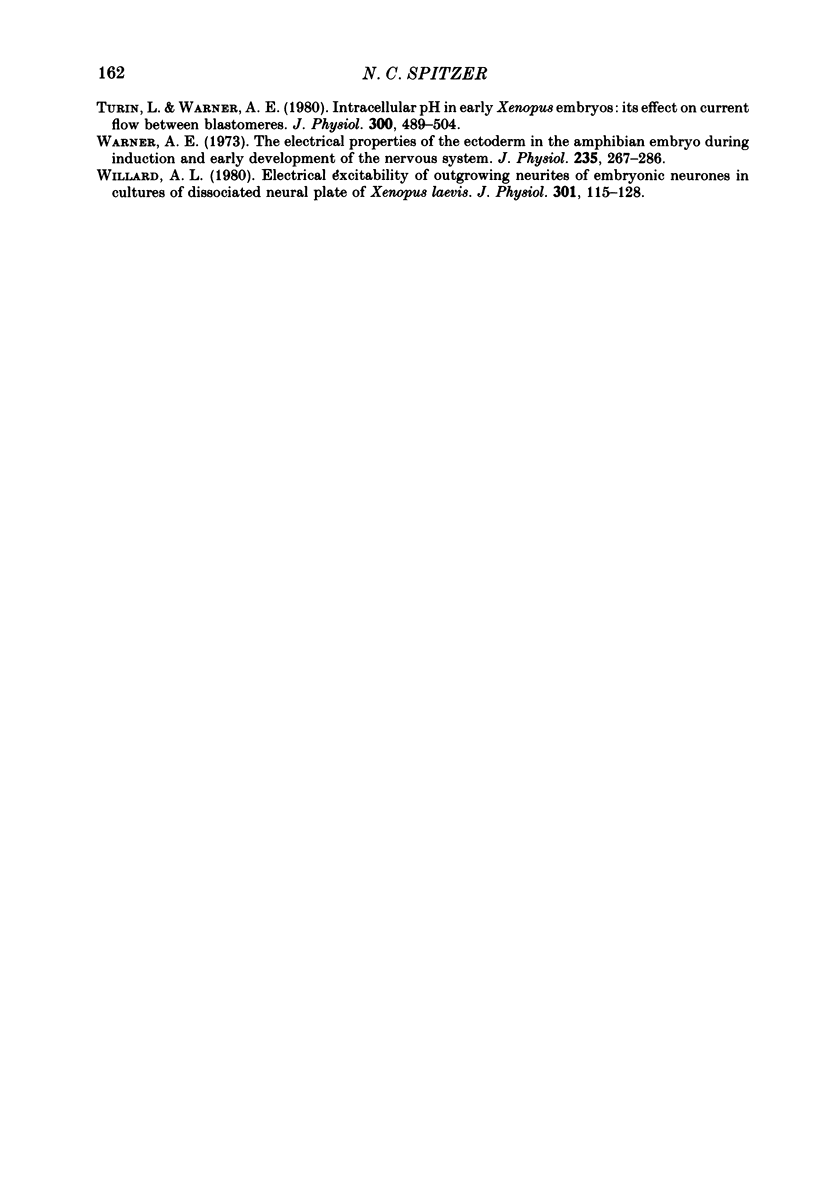
Selected References
These references are in PubMed. This may not be the complete list of references from this article.
- Baccaglini P. I., Spitzer N. C. Developmental changes in the inward current of the action potential of Rohon-Beard neurones. J Physiol. 1977 Sep;271(1):93–117. doi: 10.1113/jphysiol.1977.sp011992. [DOI] [PMC free article] [PubMed] [Google Scholar]
- Blackshaw S. E., Warner A. E. Low resistance junctions between mesoderm cells during development of trunk muscles. J Physiol. 1976 Feb;255(1):209–230. doi: 10.1113/jphysiol.1976.sp011276. [DOI] [PMC free article] [PubMed] [Google Scholar]
- Goodman C. S., Spitzer N. C. Embryonic development of identified neurones: differentiation from neuroblast to neurone. Nature. 1979 Jul 19;280(5719):208–214. doi: 10.1038/280208a0. [DOI] [PubMed] [Google Scholar]
- Goodman C. S., Spitzer N. C. The development of electrical properties of identified neurones in grasshopper embryos. J Physiol. 1981;313:385–403. doi: 10.1113/jphysiol.1981.sp013672. [DOI] [PMC free article] [PubMed] [Google Scholar]
- Hagiwara S., Takahashi K. The anomalous rectification and cation selectivity of the membrane of a starfish egg cell. J Membr Biol. 1974;18(1):61–80. doi: 10.1007/BF01870103. [DOI] [PubMed] [Google Scholar]
- Hamilton L. The formation of somites in Xenopus. J Embryol Exp Morphol. 1969 Sep;22(2):253–264. [PubMed] [Google Scholar]
- Jacobson M. Rohon-Beard neuron origin from blastomeres of the 16-cell frog embryo. J Neurosci. 1981 Aug;1(8):918–922. doi: 10.1523/JNEUROSCI.01-08-00918.1981. [DOI] [PMC free article] [PubMed] [Google Scholar]
- Lamborghini J. E., Revenaugh M., Spitzer N. C. Ultrastructural development of Rohon-Beard neurons: loss of intramitochondrial granules parallels loss of calcium action potentials. J Comp Neurol. 1979 Feb 15;183(4):741–752. doi: 10.1002/cne.901830405. [DOI] [PubMed] [Google Scholar]
- Lamborghini J. E. Rohon-beard cells and other large neurons in Xenopus embryos originate during gastrulation. J Comp Neurol. 1980 Jan 15;189(2):323–333. doi: 10.1002/cne.901890208. [DOI] [PubMed] [Google Scholar]
- Miyazaki S. I., Ohmori H., Sasaki S. Potassium rectifications of the starfish oocyte membrane and their changes during oocyte maturation. J Physiol. 1975 Mar;246(1):55–78. doi: 10.1113/jphysiol.1975.sp010880. [DOI] [PMC free article] [PubMed] [Google Scholar]
- Miyazaki S. I., Takahashi K., Tsuda K., Yoshii M. Analysis of non-linearity observed in the current-voltage relation of the tunicate embryo. J Physiol. 1974 Apr;238(1):55–77. doi: 10.1113/jphysiol.1974.sp010510. [DOI] [PMC free article] [PubMed] [Google Scholar]
- Potter D. D., Furshpan E. J., Lennox E. S. Connections between cells of the developing squid as revealed by electrophysiological methods. Proc Natl Acad Sci U S A. 1966 Feb;55(2):328–336. doi: 10.1073/pnas.55.2.328. [DOI] [PMC free article] [PubMed] [Google Scholar]
- Roberts A., Hayes B. P. The anatomy and function of 'free' nerve endings in an amphibian skin sensory system. Proc R Soc Lond B Biol Sci. 1977 Apr;196(1125):415–429. doi: 10.1098/rspb.1977.0048. [DOI] [PubMed] [Google Scholar]
- Rose B., Loewenstein W. R. Permeability of cell junction depends on local cytoplasmic calcium activity. Nature. 1975 Mar 20;254(5497):250–252. doi: 10.1038/254250a0. [DOI] [PubMed] [Google Scholar]
- Rose B., Rick R. Intracellular pH, intracellular free Ca, and junctional cell-cell coupling. J Membr Biol. 1978 Dec 29;44(3-4):377–415. doi: 10.1007/BF01944230. [DOI] [PubMed] [Google Scholar]
- Sheridan J. D. Electrophysiological evidence for low-resistance intercellular junctions in the early chick embryo. J Cell Biol. 1968 Jun;37(3):650–659. doi: 10.1083/jcb.37.3.650. [DOI] [PMC free article] [PubMed] [Google Scholar]
- Spitzer N. C., Lamborghini J. E. The development of the action potential mechanism of amphibian neurons isolated in culture. Proc Natl Acad Sci U S A. 1976 May;73(5):1641–1645. doi: 10.1073/pnas.73.5.1641. [DOI] [PMC free article] [PubMed] [Google Scholar]
- Spitzer N. C. Low pH selectively blocks calcium action potentials in amphibian neurons developing in culture. Brain Res. 1979 Feb 9;161(3):555–559. doi: 10.1016/0006-8993(79)90687-5. [DOI] [PubMed] [Google Scholar]
- Spitzer N. C. Low resistance connections between cells in the developing anther of the lily. J Cell Biol. 1970 Jun;45(3):565–575. doi: 10.1083/jcb.45.3.565. [DOI] [PMC free article] [PubMed] [Google Scholar]
- Spitzer N. C. The ionic basis of the resting potential and a slow depolarizing response in Rohon-Beard neurones of Xenopus tadpoles. J Physiol. 1976 Feb;255(1):105–135. doi: 10.1113/jphysiol.1976.sp011272. [DOI] [PMC free article] [PubMed] [Google Scholar]
- Spray D. C., Harris A. L., Bennett M. V. Equilibrium properties of a voltage-dependent junctional conductance. J Gen Physiol. 1981 Jan;77(1):77–93. doi: 10.1085/jgp.77.1.77. [DOI] [PMC free article] [PubMed] [Google Scholar]
- Spray D. C., Harris A. L., Bennett M. V. Gap junctional conductance is a simple and sensitive function of intracellular pH. Science. 1981 Feb 13;211(4483):712–715. doi: 10.1126/science.6779379. [DOI] [PubMed] [Google Scholar]
- Spray D. C., Harris A. L., Bennett M. V. Voltage dependence of junctional conductance in early amphibian embryos. Science. 1979 Apr 27;204(4391):432–434. doi: 10.1126/science.312530. [DOI] [PubMed] [Google Scholar]
- Turin L., Warner A. E. Intracellular pH in early Xenopus embryos: its effect on current flow between blastomeres. J Physiol. 1980 Mar;300:489–504. doi: 10.1113/jphysiol.1980.sp013174. [DOI] [PMC free article] [PubMed] [Google Scholar]
- Turin L., Warner A. Carbon dioxide reversibly abolishes ionic communication between cells of early amphibian embryo. Nature. 1977 Nov 3;270(5632):56–57. doi: 10.1038/270056a0. [DOI] [PubMed] [Google Scholar]
- Warner A. E. The electrical properties of the ectoderm in the amphibian embryo during induction and early development of the nervous system. J Physiol. 1973 Nov;235(1):267–286. doi: 10.1113/jphysiol.1973.sp010387. [DOI] [PMC free article] [PubMed] [Google Scholar]
- Willard A. L. Electrical excitability of outgrowing neurites of embryonic neurones in cultures of dissociated neural plate of Xenopus laevis. J Physiol. 1980 Apr;301:115–128. doi: 10.1113/jphysiol.1980.sp013193. [DOI] [PMC free article] [PubMed] [Google Scholar]