Abstract
We present a detailed computational study of the influence of salt on the configurations, energies, and dynamics of supercoiled DNA. A potential function that includes both elastic and electrostatic energy components is employed. Specifically, the electrostatic term, with salt-dependent coefficients, is modeled after Stigter's pioneering work on the effective diameter of DNA as a function of salt concentration. Because an effective charge per unit length is used, the electrostatic formulation does not require explicit modeling of phosphates and can be used to study long DNAs at any desired resolution of charge. With explicit consideration of the electrostatic energy, an elastic bending constant corresponding to the nonelectrostatic part of the bending contribution to the persistence length is used. We show, for a series of salt concentrations ranging from 0.005 to 1.0 M sodium, how configurations and energies of supercoiled DNA (1000 and 3000 base pairs) change dramatically with the simulated salt environment. At high salt, the DNA adopts highly compact and bent interwound states, with the bending energy dominating over the other components, and the electrostatic energy playing a minor role in comparison to the bending and twisting terms. At low salt, the DNA supercoils are much more open and loosely interwound, and the electrostatic components are dominant. Over the range of three decades of salt examined, the electrostatic energy changes by a factor of 10. The buckling transition between the circle and figure-8 is highly sensitive to salt concentration: this transition is delayed as salt concentration decreases, with a particularly sharp increase below 0.1 M. For example, for a bending-to-twisting force constant ratio of A/C = 1.5, the linking number difference (delta LK) corresponding to equal energies for the circle and figure-8 increases from 2.1 to 3.25 as salt decreases from 1.0 to 0.005 M. We also present in detail a family of three-lobed supercoiled DNA configurations that are predicted by elasticity theory to be stable at low delta Lk. To our knowledge, such three-dimensional structures have not been previously presented in connection with DNA supercoiling. These branched forms have a higher bending energy than the corresponding interwound configurations at the same delta Lk but, especially at low salt, this bending energy difference is relatively small in comparison with the total energy, which is dominated by the electrostatic contributions. Significantly, the electrostatic energies of the three-lobed and (straight) interwound forms are comparable at each salt environment. We show how the three-lobed configurations change slowly with ALk, resulting in branched interwound forms at higher salt. In longer chains, the branched forms are highly interwound, with bent arms. At low salt, the branched supercoils are asymmetric, with a longer interwound stem and two shorter arms. From molecular dynamics simulations we observe differences in the motions of the DNA as a function of salt. At high salt, the supercoiled chain is quite compact but fairly rigid, whereas at low salt the DNA is loosely coiled but more dynamic. Especially notable at low salt are the large-scale opening and closing of the chain as a whole and the rapid "slithering"of individual residues past one another. Toroidal forms are not detected under these conditions. However, the overall features of the open, loose supercoils found at low salt are more similar to those of toroidal than interwound configurations. Indeed,simulated x-ray scattering profiles reveal the same trends observed experimentally and are consistent with a change from closed to open forms as salt is decreased. Like the minimization studies, the dynamics reveal a critical point near 0.1 M associated with the collapse of loose to tight supercoils. Near this physiological concentration, enhanced flexibility of the DNA is noted. The collective observations suggest a potential regulatory role for salt on supercoiled DNA function, not only for closed circular DNA,but also for linear DNA with small looped regions.
Full text
PDF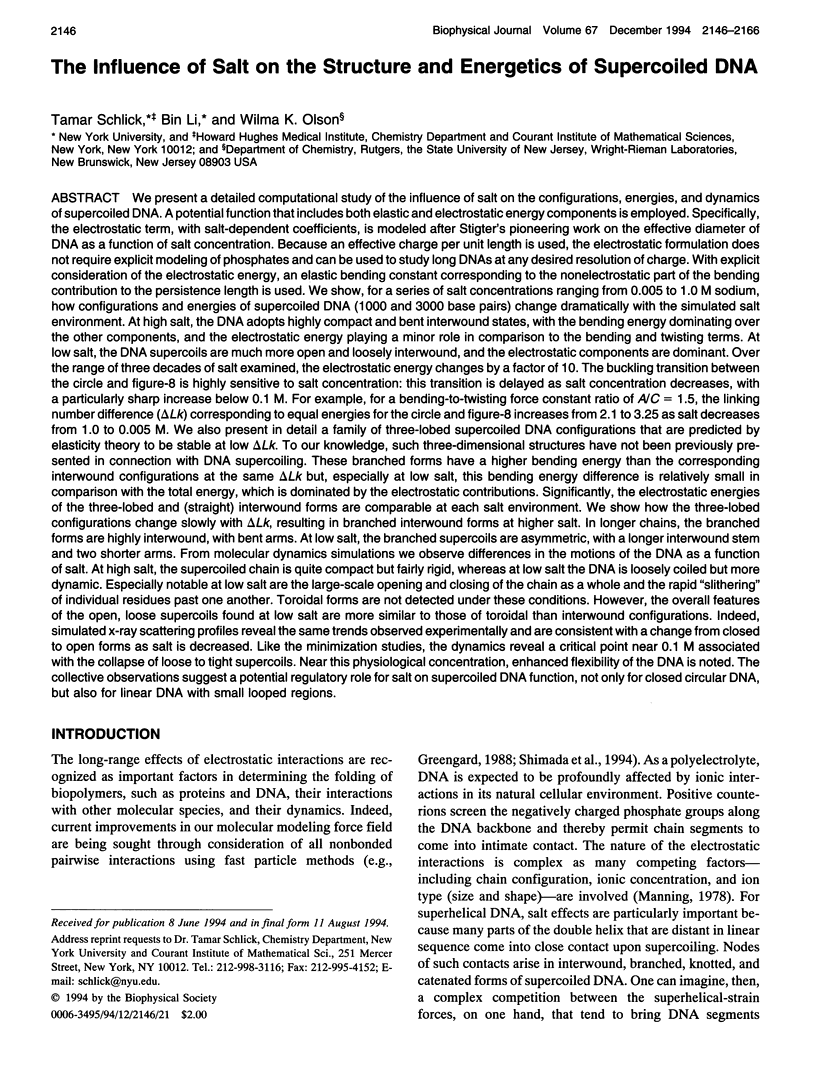
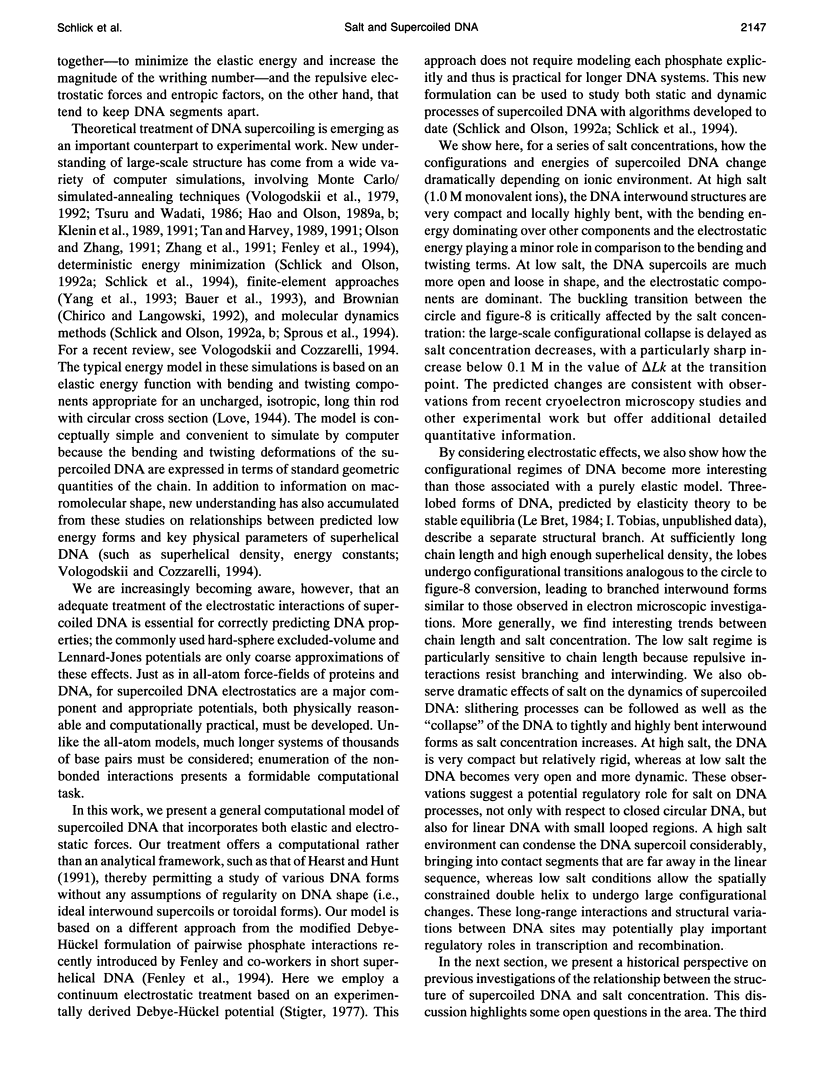
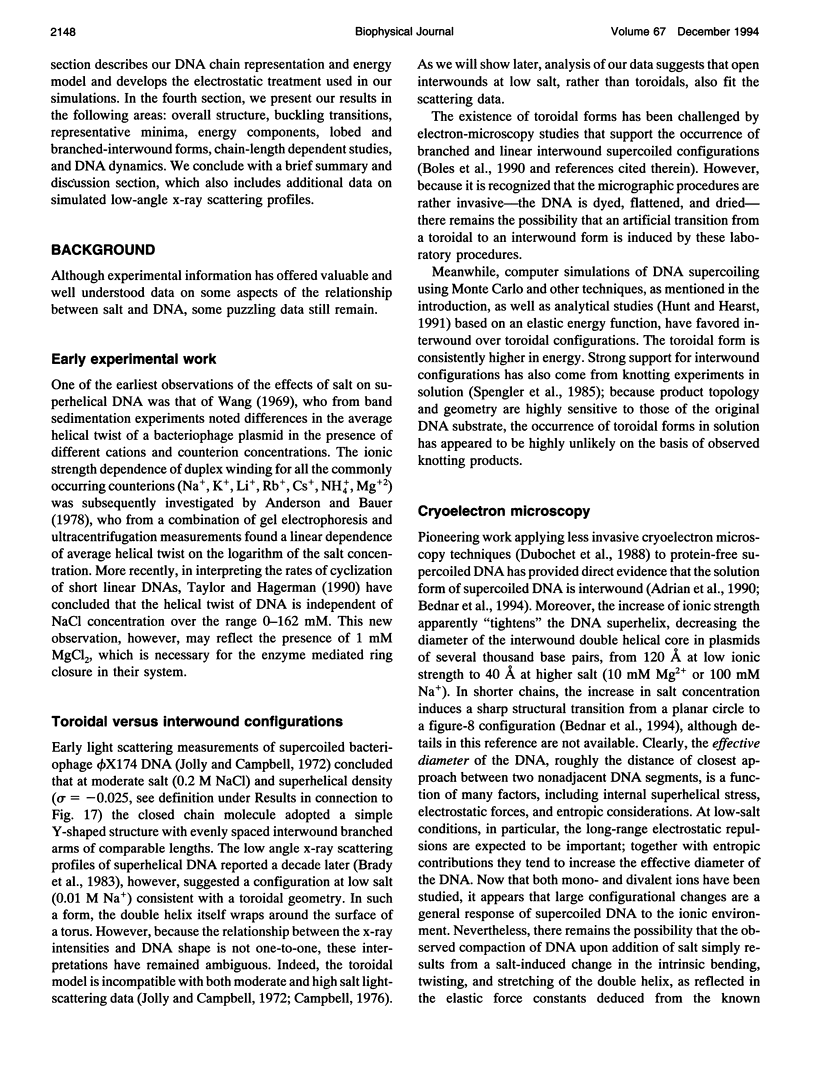
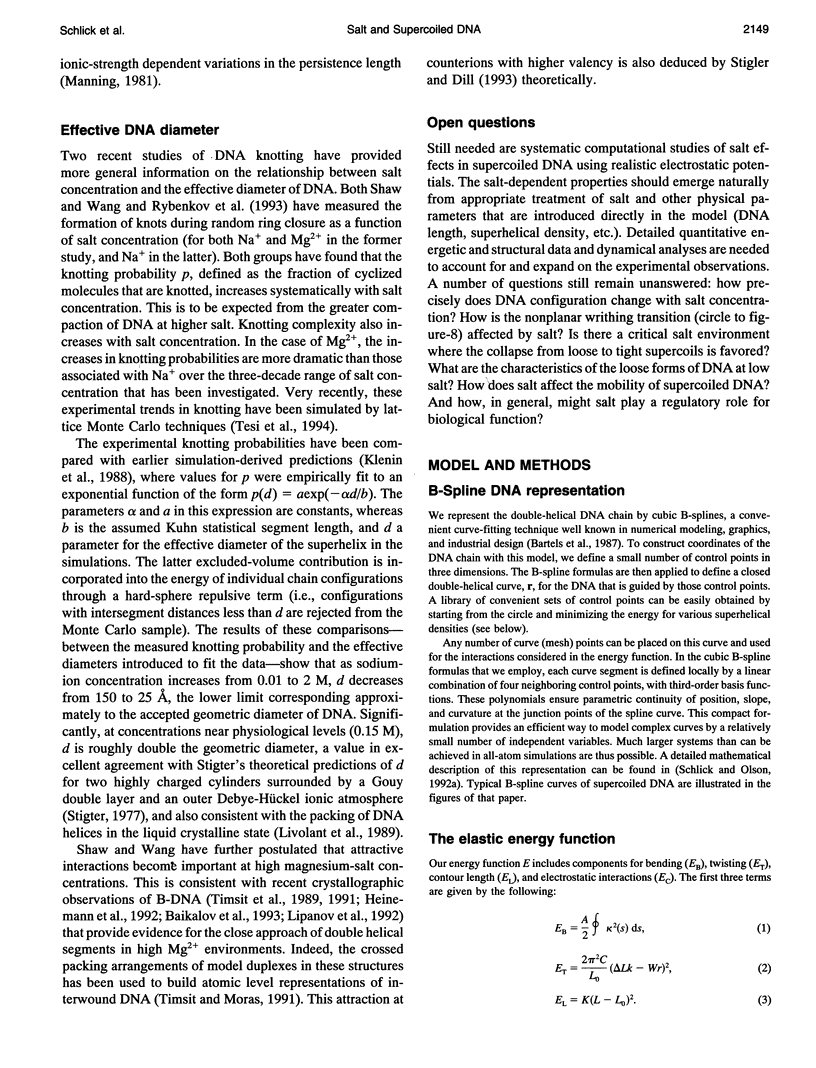
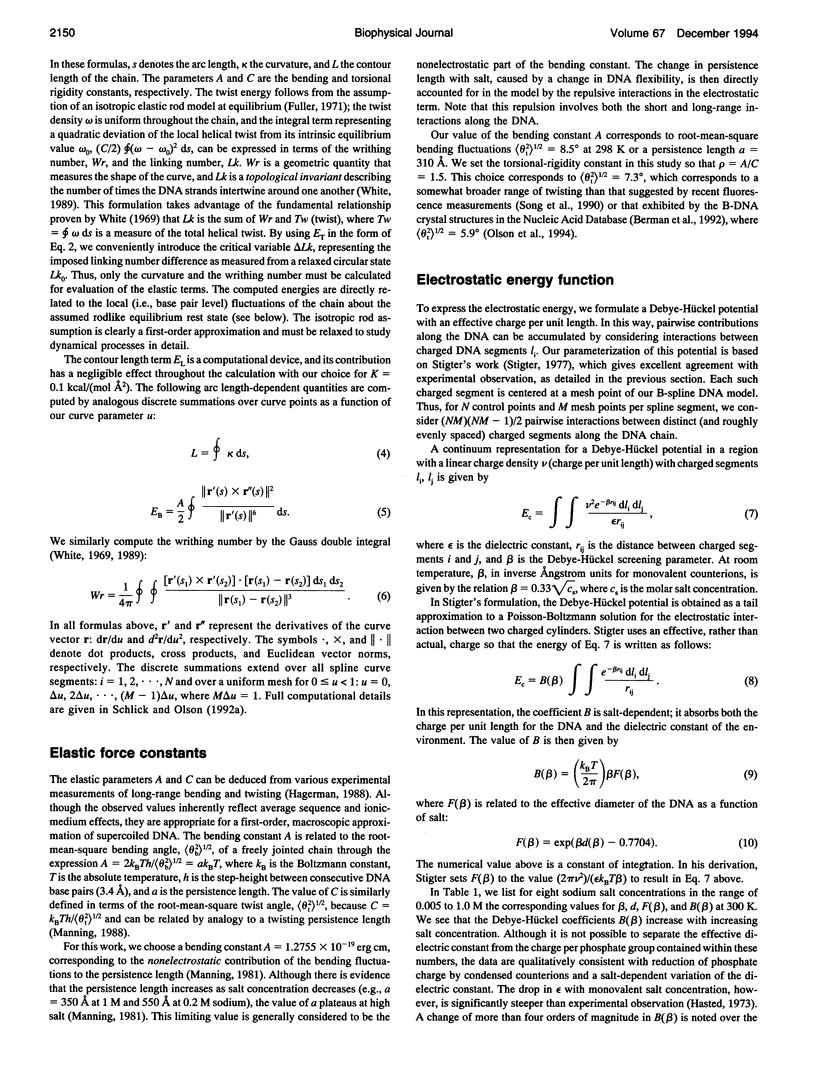
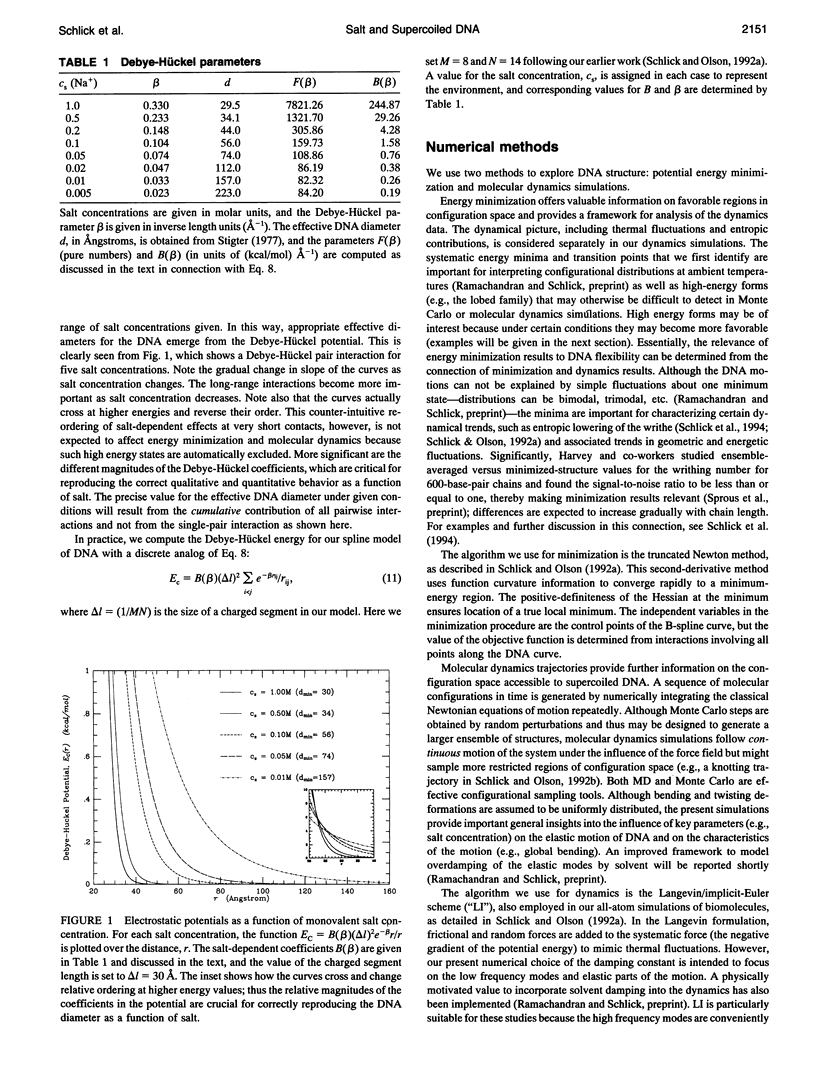
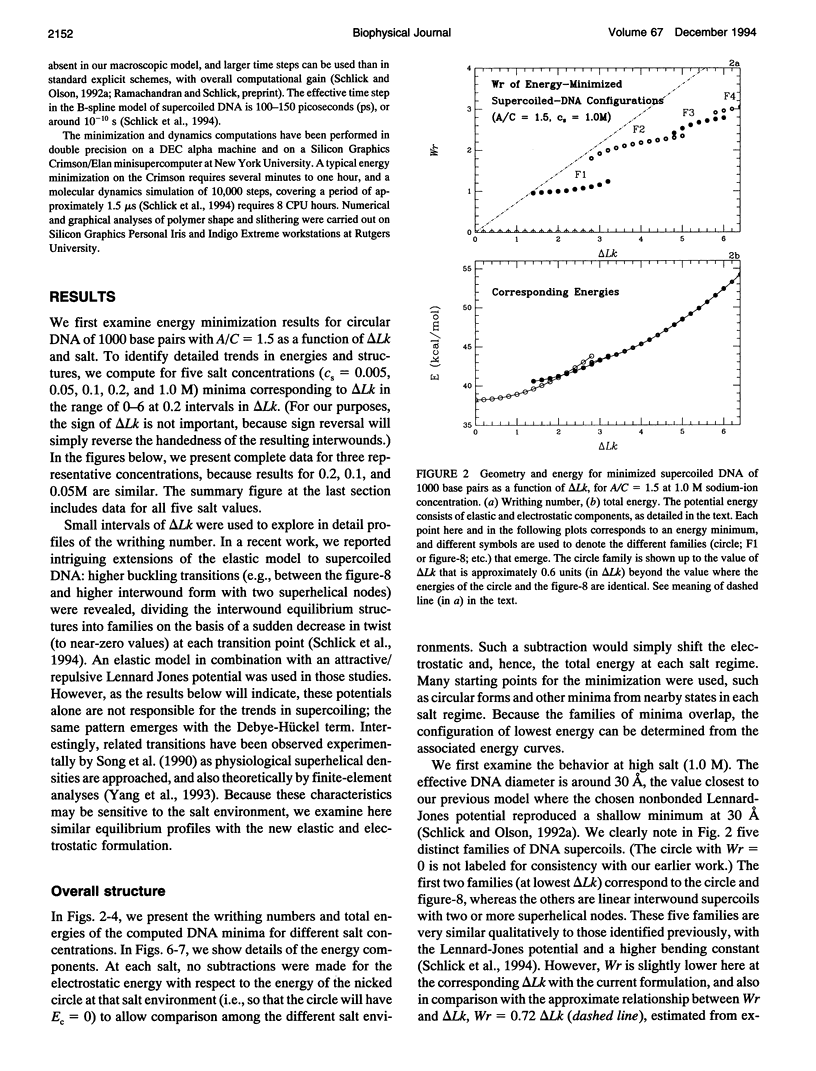
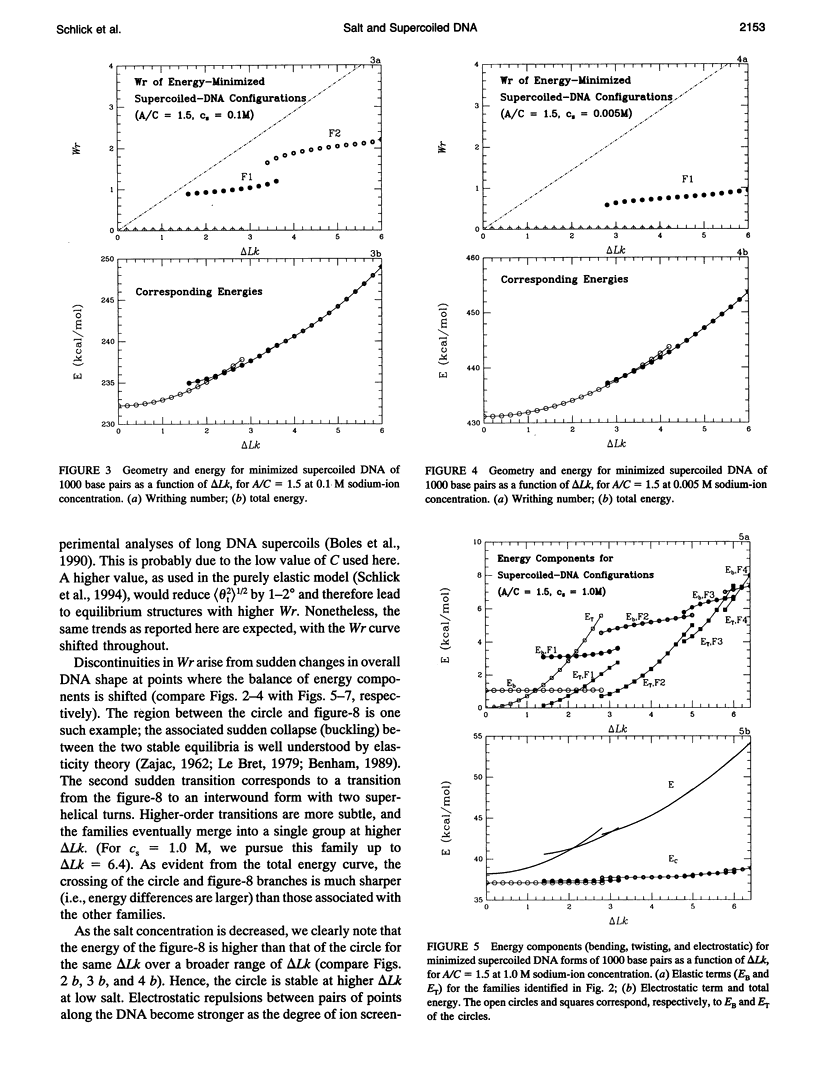
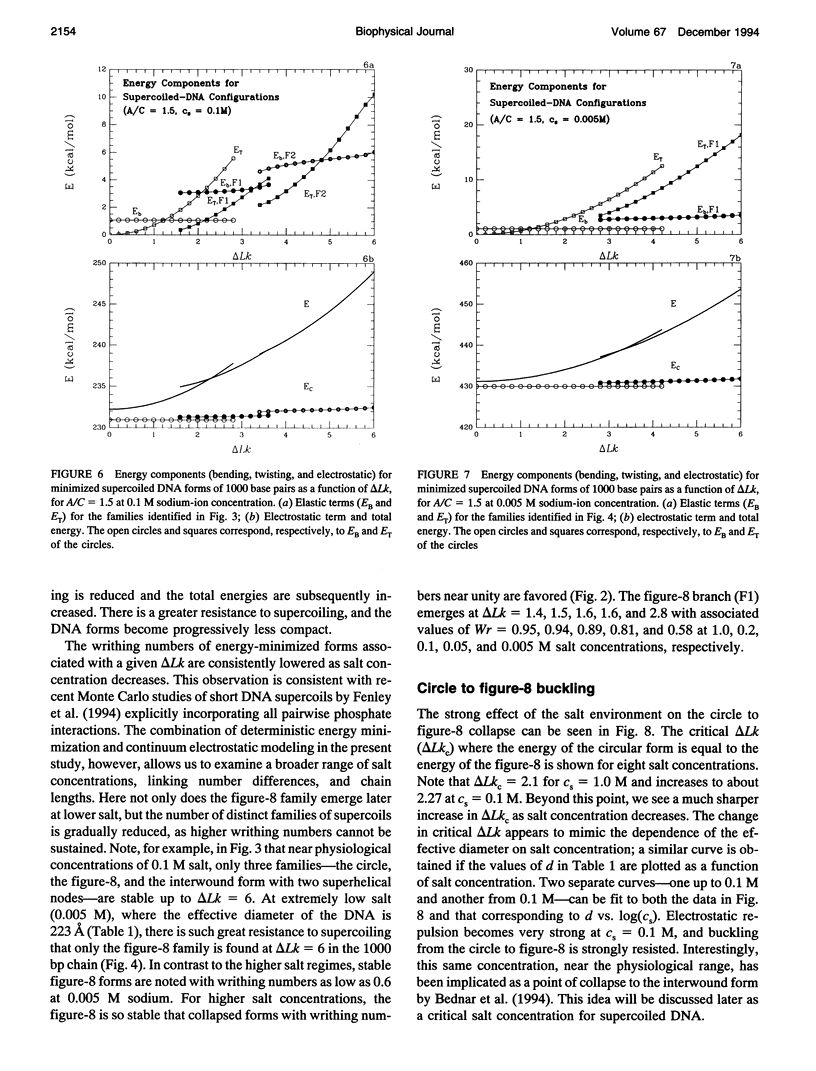
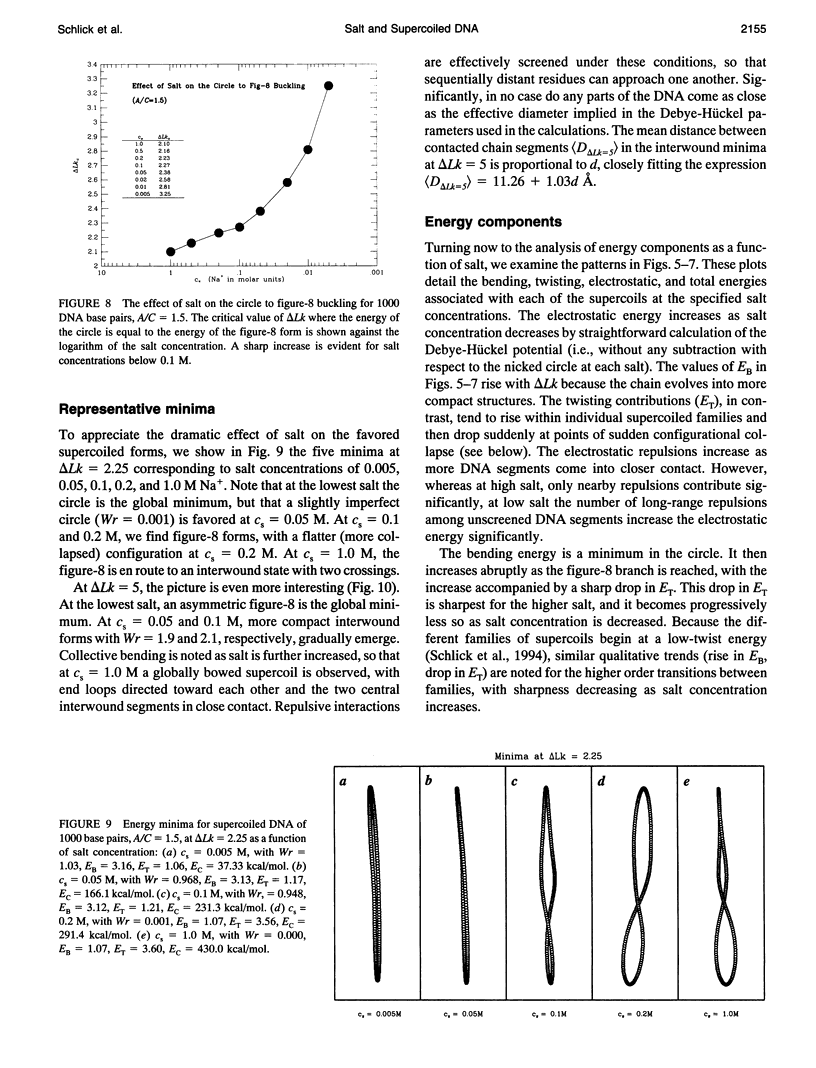
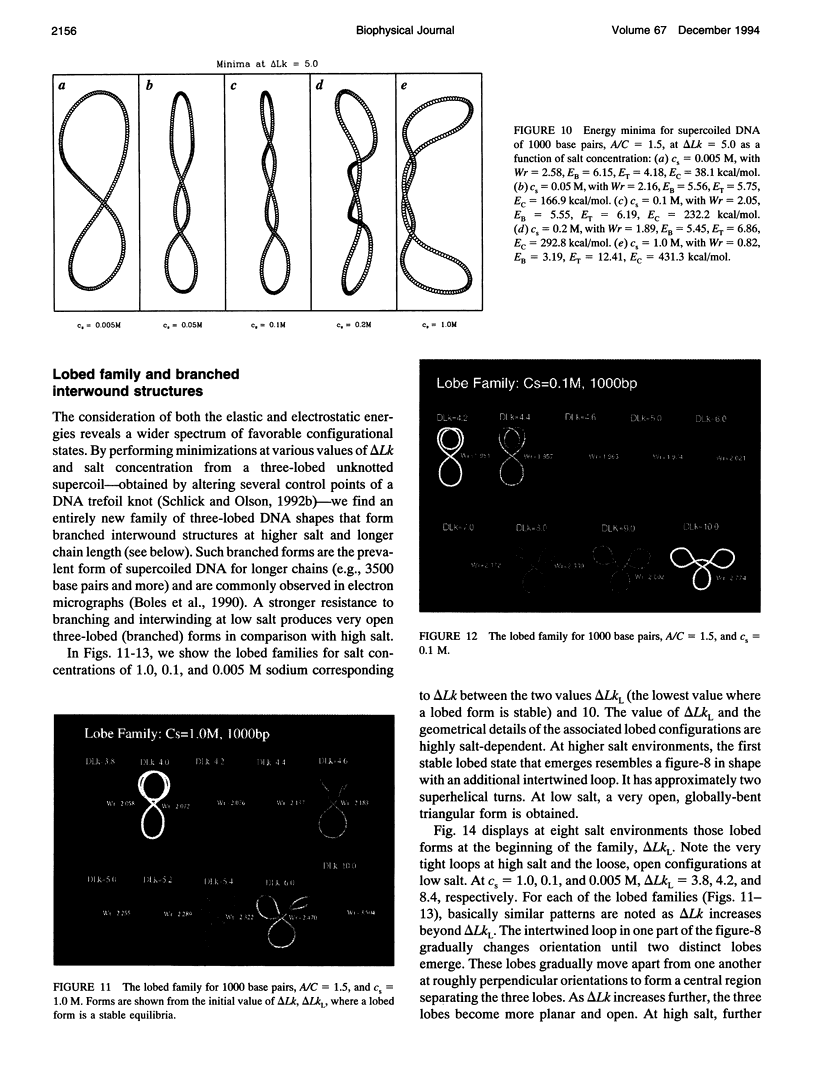
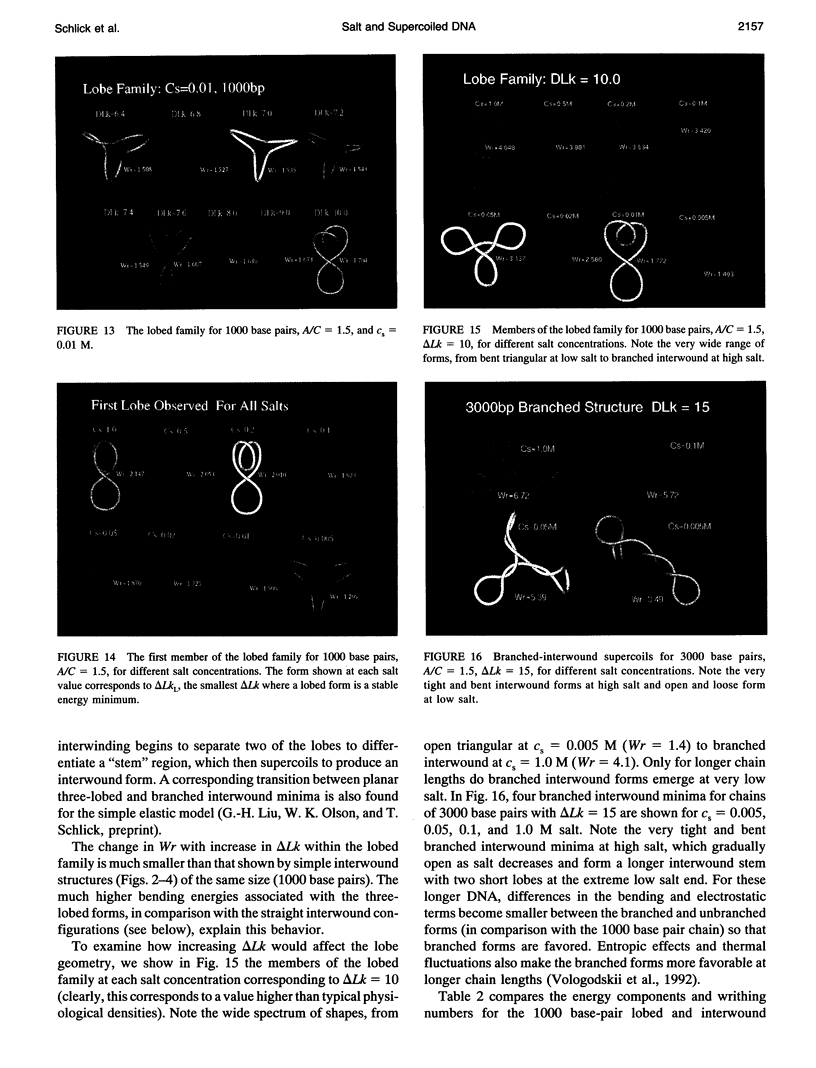
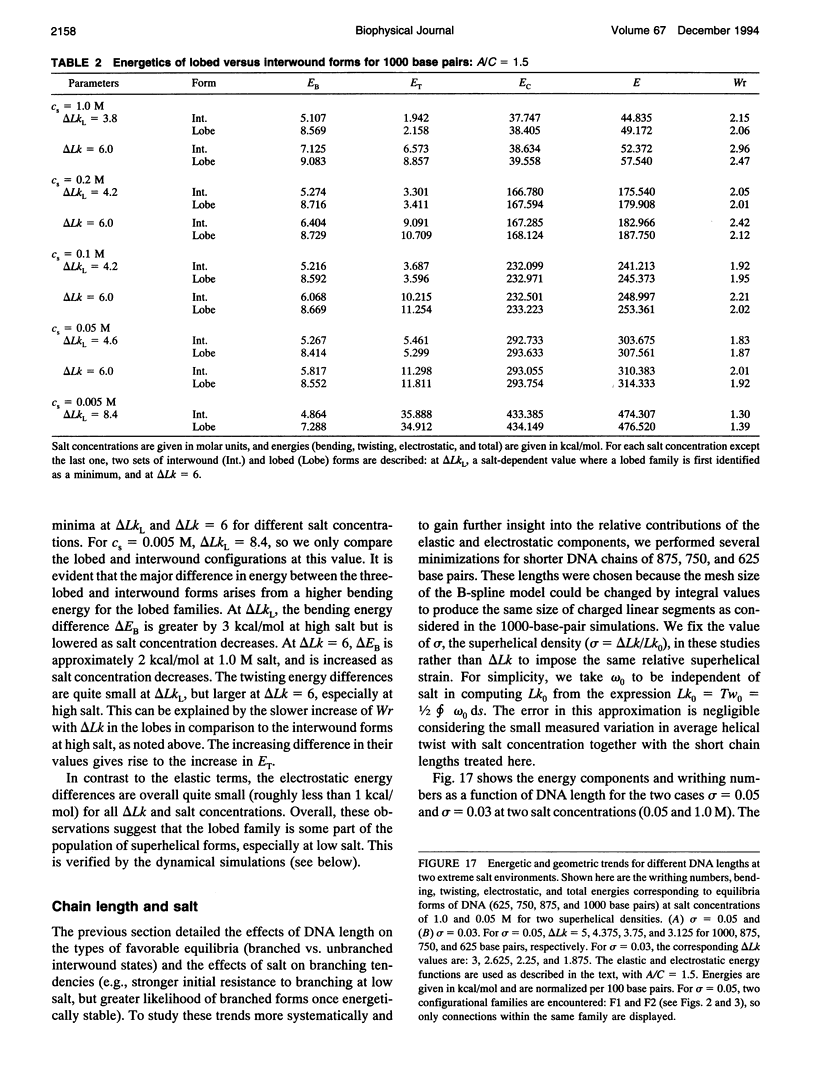
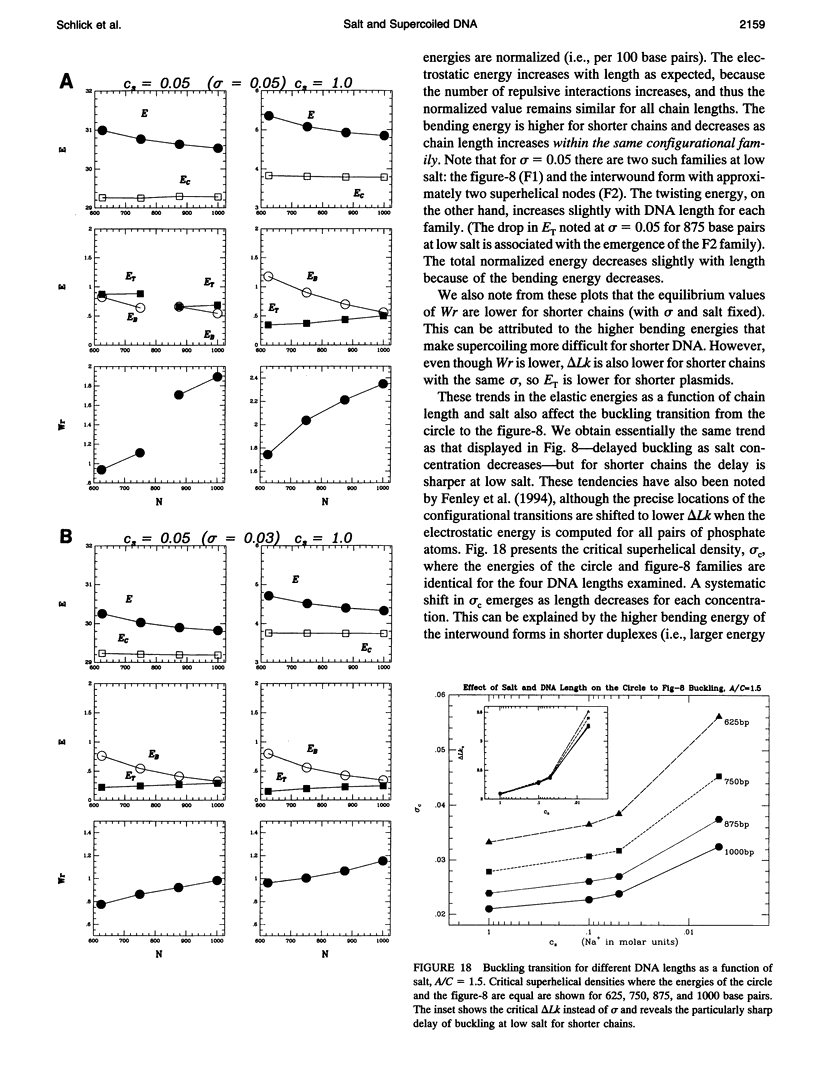
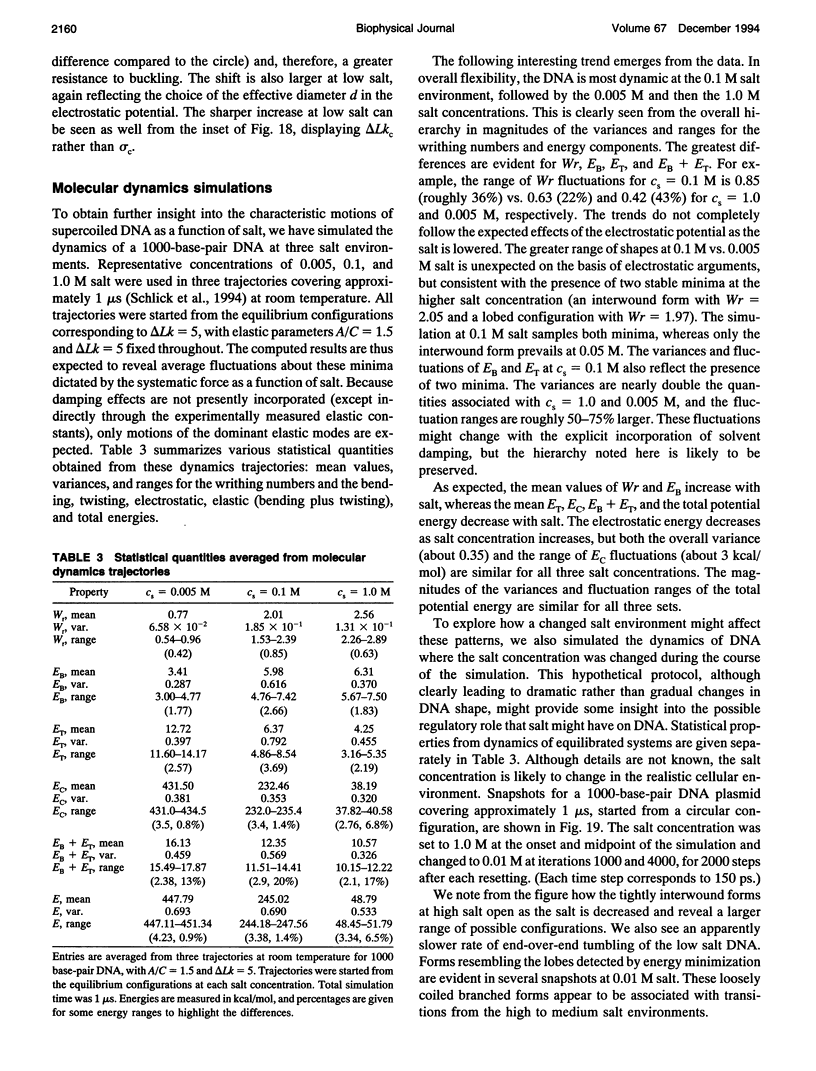
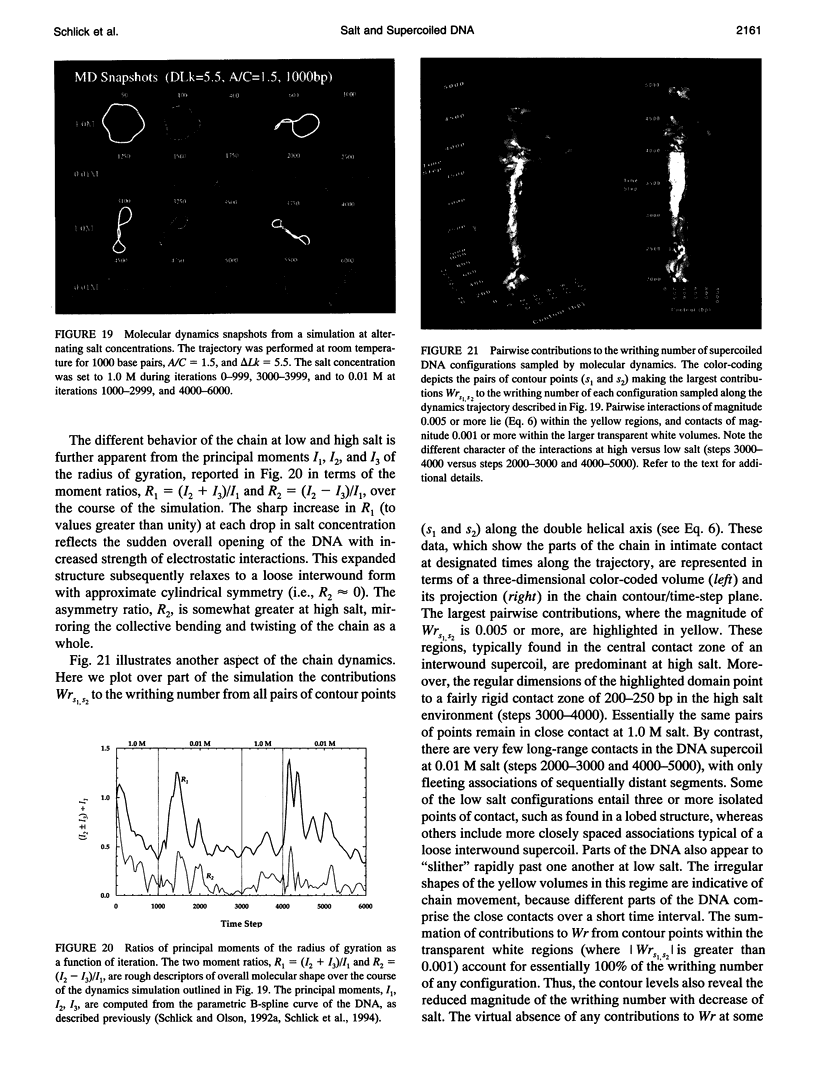
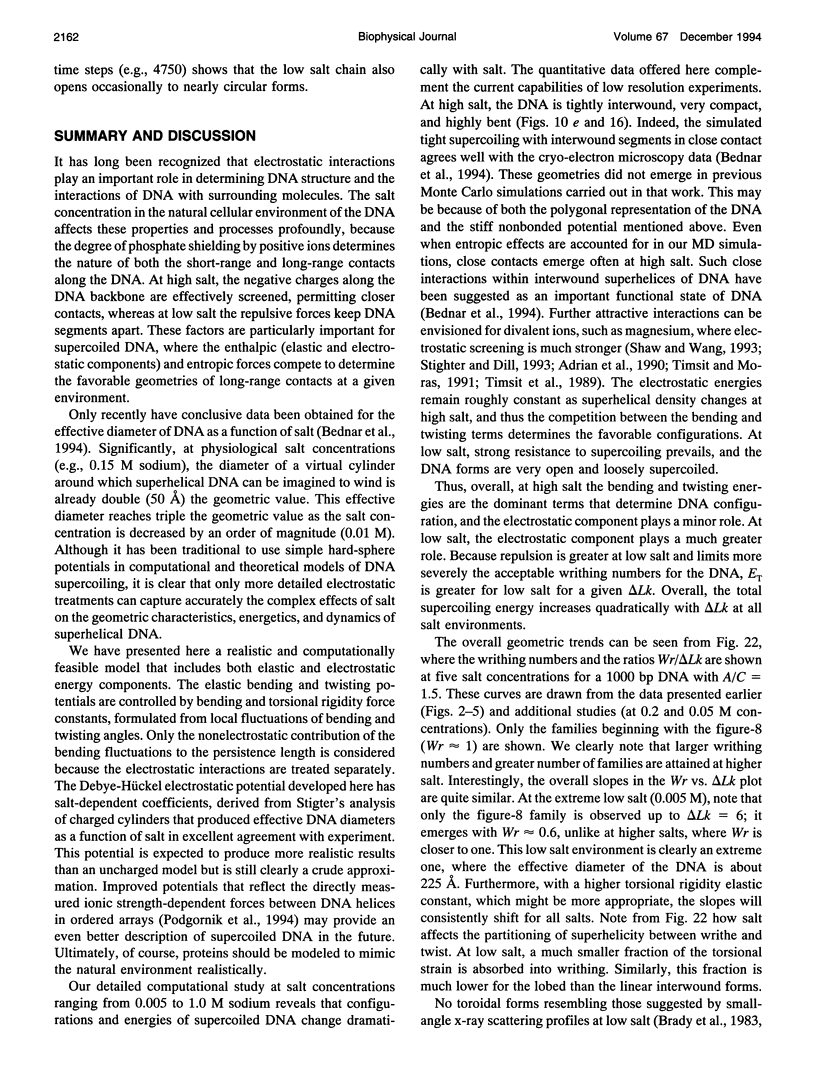
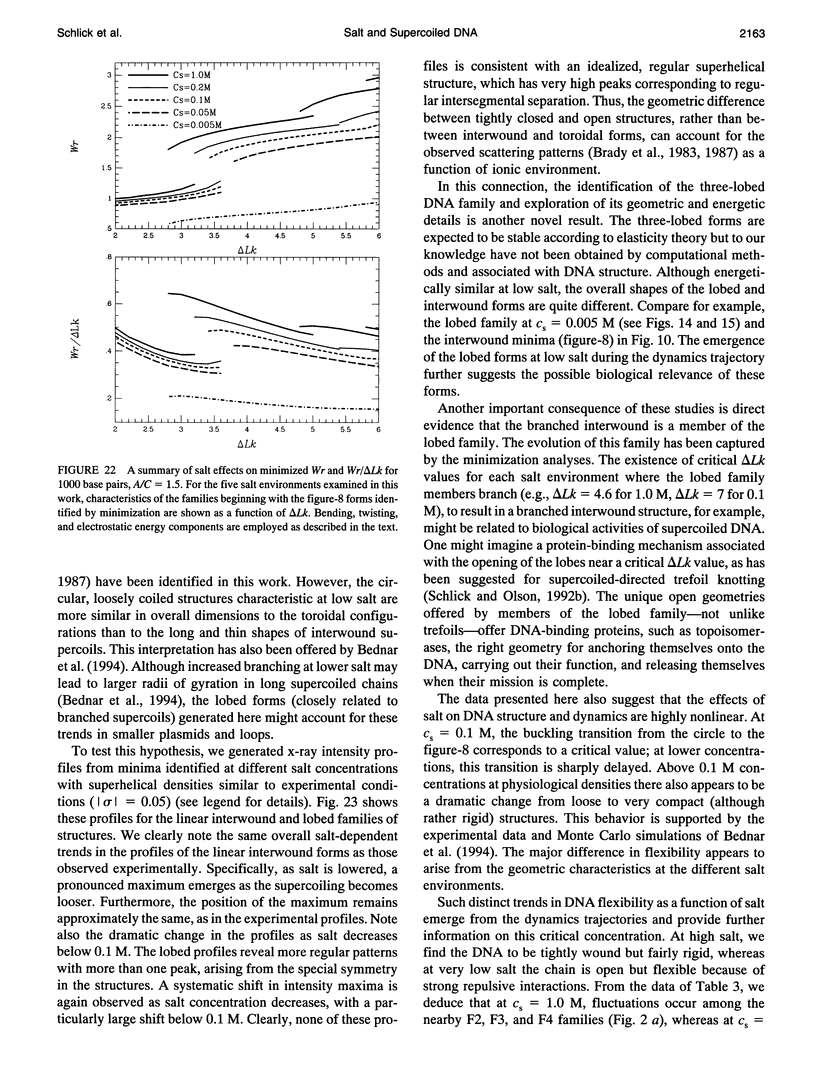
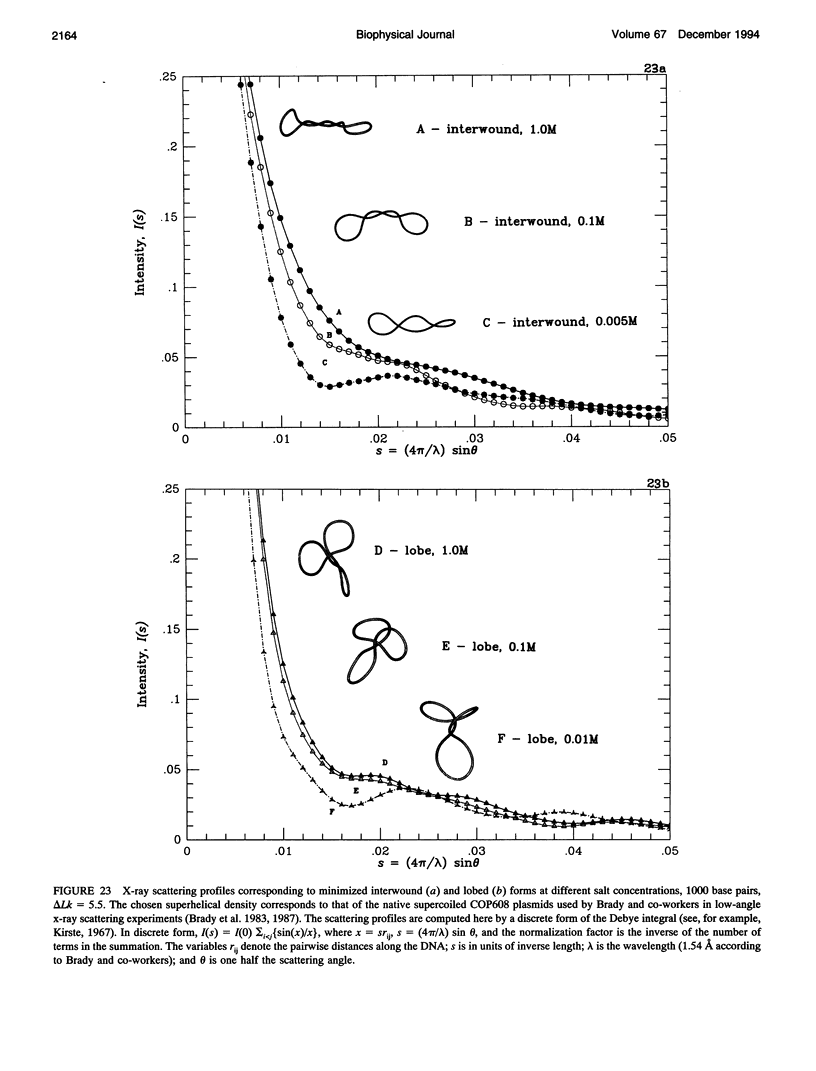
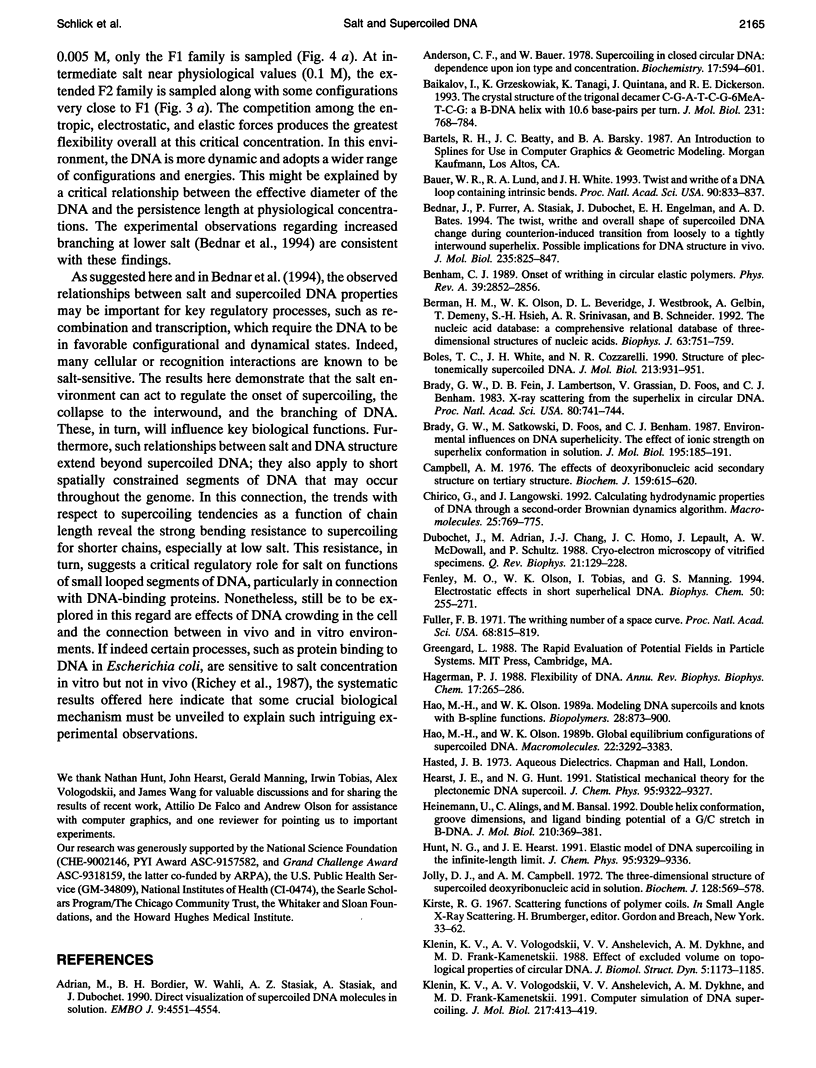
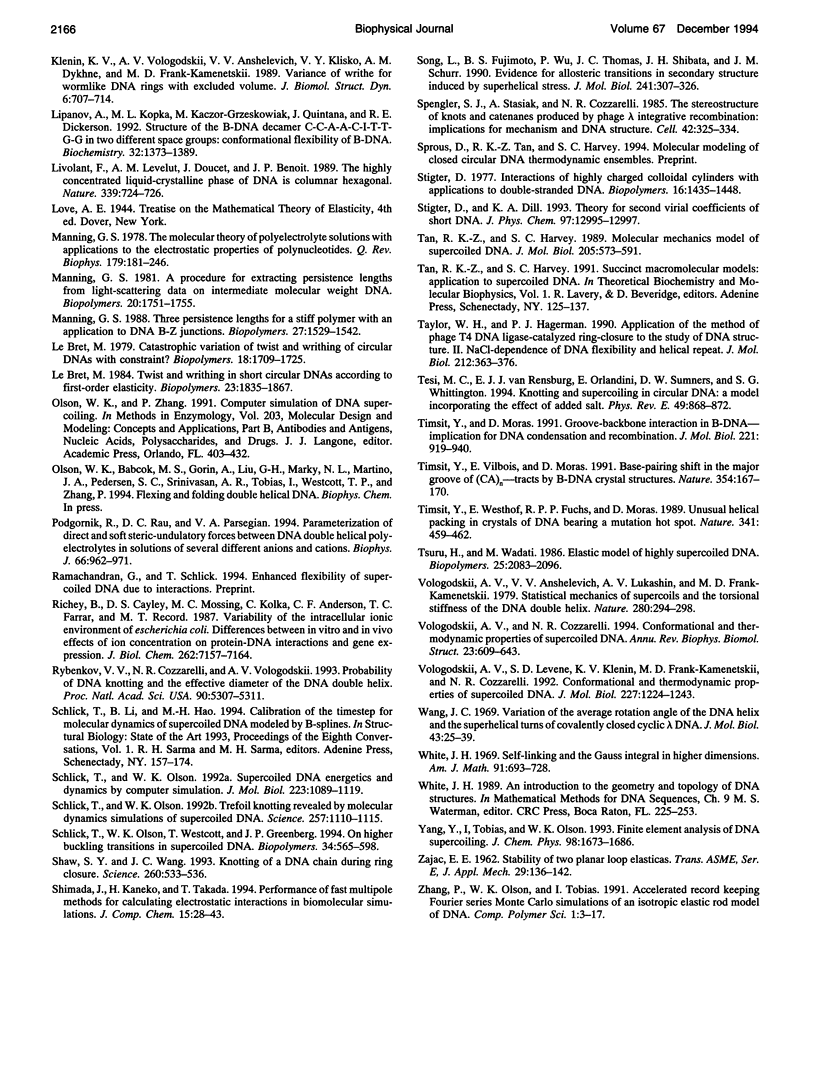
Images in this article
Selected References
These references are in PubMed. This may not be the complete list of references from this article.
- Adrian M., ten Heggeler-Bordier B., Wahli W., Stasiak A. Z., Stasiak A., Dubochet J. Direct visualization of supercoiled DNA molecules in solution. EMBO J. 1990 Dec;9(13):4551–4554. doi: 10.1002/j.1460-2075.1990.tb07907.x. [DOI] [PMC free article] [PubMed] [Google Scholar]
- Anderson P., Bauer W. Supercoiling in closed circular DNA: dependence upon ion type and concentration. Biochemistry. 1978 Feb 21;17(4):594–601. doi: 10.1021/bi00597a006. [DOI] [PubMed] [Google Scholar]
- Baikalov I., Grzeskowiak K., Yanagi K., Quintana J., Dickerson R. E. The crystal structure of the trigonal decamer C-G-A-T-C-G-6meA-T-C-G: a B-DNA helix with 10.6 base-pairs per turn. J Mol Biol. 1993 Jun 5;231(3):768–784. doi: 10.1006/jmbi.1993.1325. [DOI] [PubMed] [Google Scholar]
- Bauer W. R., Lund R. A., White J. H. Twist and writhe of a DNA loop containing intrinsic bends. Proc Natl Acad Sci U S A. 1993 Feb 1;90(3):833–837. doi: 10.1073/pnas.90.3.833. [DOI] [PMC free article] [PubMed] [Google Scholar]
- Bednar J., Furrer P., Stasiak A., Dubochet J., Egelman E. H., Bates A. D. The twist, writhe and overall shape of supercoiled DNA change during counterion-induced transition from a loosely to a tightly interwound superhelix. Possible implications for DNA structure in vivo. J Mol Biol. 1994 Jan 21;235(3):825–847. doi: 10.1006/jmbi.1994.1042. [DOI] [PubMed] [Google Scholar]
- Berman H. M., Olson W. K., Beveridge D. L., Westbrook J., Gelbin A., Demeny T., Hsieh S. H., Srinivasan A. R., Schneider B. The nucleic acid database. A comprehensive relational database of three-dimensional structures of nucleic acids. Biophys J. 1992 Sep;63(3):751–759. doi: 10.1016/S0006-3495(92)81649-1. [DOI] [PMC free article] [PubMed] [Google Scholar]
- Boles T. C., White J. H., Cozzarelli N. R. Structure of plectonemically supercoiled DNA. J Mol Biol. 1990 Jun 20;213(4):931–951. doi: 10.1016/S0022-2836(05)80272-4. [DOI] [PubMed] [Google Scholar]
- Brady G. W., Fein D. B., Lambertson H., Grassian V., Foos D., Benham C. J. X-ray scattering from the superhelix in circular DNA. Proc Natl Acad Sci U S A. 1983 Feb;80(3):741–744. doi: 10.1073/pnas.80.3.741. [DOI] [PMC free article] [PubMed] [Google Scholar]
- Brady G. W., Satkowski M., Foos D., Benham C. J. Environmental influences on DNA superhelicity. The effect of ionic strength on superhelix conformation in solution. J Mol Biol. 1987 May 5;195(1):185–191. doi: 10.1016/0022-2836(87)90335-4. [DOI] [PubMed] [Google Scholar]
- Campbell A. M. The effects of deoxyribonucleic acid secondary structure on tertiary structure. Biochem J. 1976 Dec 1;159(3):615–620. doi: 10.1042/bj1590615. [DOI] [PMC free article] [PubMed] [Google Scholar]
- Dubochet J., Adrian M., Chang J. J., Homo J. C., Lepault J., McDowall A. W., Schultz P. Cryo-electron microscopy of vitrified specimens. Q Rev Biophys. 1988 May;21(2):129–228. doi: 10.1017/s0033583500004297. [DOI] [PubMed] [Google Scholar]
- Fenley M. O., Olson W. K., Tobias I., Manning G. S. Electrostatic effects in short superhelical DNA. Biophys Chem. 1994 Jun;50(3):255–271. doi: 10.1016/0301-4622(93)e0094-l. [DOI] [PubMed] [Google Scholar]
- Fuller F. B. The writhing number of a space curve. Proc Natl Acad Sci U S A. 1971 Apr;68(4):815–819. doi: 10.1073/pnas.68.4.815. [DOI] [PMC free article] [PubMed] [Google Scholar]
- Hagerman P. J. Flexibility of DNA. Annu Rev Biophys Biophys Chem. 1988;17:265–286. doi: 10.1146/annurev.bb.17.060188.001405. [DOI] [PubMed] [Google Scholar]
- Hao M. H., Olson W. K. Modeling DNA supercoils and knots with B-spline functions. Biopolymers. 1989 Apr;28(4):873–900. doi: 10.1002/bip.360280407. [DOI] [PubMed] [Google Scholar]
- Heinemann U., Alings C. Crystallographic study of one turn of G/C-rich B-DNA. J Mol Biol. 1989 Nov 20;210(2):369–381. doi: 10.1016/0022-2836(89)90337-9. [DOI] [PubMed] [Google Scholar]
- Jolly D. J., Campbell A. M. The three-dimensional structure of supercoiled deoxyribonucleic acid in solution. Evidence obtained from the angular distribution of scattered light. Biochem J. 1972 Jul;128(3):569–578. doi: 10.1042/bj1280569. [DOI] [PMC free article] [PubMed] [Google Scholar]
- Klenin K. V., Vologodskii A. V., Anshelevich V. V., Dykhne A. M., Frank-Kamenetskii M. D. Computer simulation of DNA supercoiling. J Mol Biol. 1991 Feb 5;217(3):413–419. doi: 10.1016/0022-2836(91)90745-r. [DOI] [PubMed] [Google Scholar]
- Klenin K. V., Vologodskii A. V., Anshelevich V. V., Dykhne A. M., Frank-Kamenetskii M. D. Effect of excluded volume on topological properties of circular DNA. J Biomol Struct Dyn. 1988 Jun;5(6):1173–1185. doi: 10.1080/07391102.1988.10506462. [DOI] [PubMed] [Google Scholar]
- Klenin K. V., Vologodskii A. V., Anshelevich V. V., Klishko VYu, Dykhne A. M., Frank-Kamenetskii M. D. Variance of writhe for wormlike DNA rings with excluded volume. J Biomol Struct Dyn. 1989 Feb;6(4):707–714. doi: 10.1080/07391102.1989.10507731. [DOI] [PubMed] [Google Scholar]
- Le Bret M. Catastrophic variation of twist and writhing of circular DNAs with constraint? Biopolymers. 1979 Jul;18(7):1709–1725. doi: 10.1002/bip.1979.360180710. [DOI] [PubMed] [Google Scholar]
- Le Bret M. Twist and writhing in short circular DNAs according to first-order elasticity. Biopolymers. 1984 Oct;23(10):1835–1867. doi: 10.1002/bip.360231004. [DOI] [PubMed] [Google Scholar]
- Lipanov A., Kopka M. L., Kaczor-Grzeskowiak M., Quintana J., Dickerson R. E. Structure of the B-DNA decamer C-C-A-A-C-I-T-T-G-G in two different space groups: conformational flexibility of B-DNA. Biochemistry. 1993 Feb 9;32(5):1373–1389. doi: 10.1021/bi00056a024. [DOI] [PubMed] [Google Scholar]
- Livolant F., Levelut A. M., Doucet J., Benoit J. P. The highly concentrated liquid-crystalline phase of DNA is columnar hexagonal. Nature. 1989 Jun 29;339(6227):724–726. doi: 10.1038/339724a0. [DOI] [PubMed] [Google Scholar]
- Manning G. S. The molecular theory of polyelectrolyte solutions with applications to the electrostatic properties of polynucleotides. Q Rev Biophys. 1978 May;11(2):179–246. doi: 10.1017/s0033583500002031. [DOI] [PubMed] [Google Scholar]
- Manning G. S. Three persistence lengths for a stiff polymer with an application to DNA B-Z junctions. Biopolymers. 1988 Oct;27(10):1529–1542. doi: 10.1002/bip.360271002. [DOI] [PubMed] [Google Scholar]
- Podgornik R., Rau D. C., Parsegian V. A. Parametrization of direct and soft steric-undulatory forces between DNA double helical polyelectrolytes in solutions of several different anions and cations. Biophys J. 1994 Apr;66(4):962–971. doi: 10.1016/S0006-3495(94)80877-X. [DOI] [PMC free article] [PubMed] [Google Scholar]
- Richey B., Cayley D. S., Mossing M. C., Kolka C., Anderson C. F., Farrar T. C., Record M. T., Jr Variability of the intracellular ionic environment of Escherichia coli. Differences between in vitro and in vivo effects of ion concentrations on protein-DNA interactions and gene expression. J Biol Chem. 1987 May 25;262(15):7157–7164. [PubMed] [Google Scholar]
- Rybenkov V. V., Cozzarelli N. R., Vologodskii A. V. Probability of DNA knotting and the effective diameter of the DNA double helix. Proc Natl Acad Sci U S A. 1993 Jun 1;90(11):5307–5311. doi: 10.1073/pnas.90.11.5307. [DOI] [PMC free article] [PubMed] [Google Scholar]
- Schlick T., Olson W. K. Supercoiled DNA energetics and dynamics by computer simulation. J Mol Biol. 1992 Feb 20;223(4):1089–1119. doi: 10.1016/0022-2836(92)90263-j. [DOI] [PubMed] [Google Scholar]
- Schlick T., Olson W. K. Trefoil knotting revealed by molecular dynamics simulations of supercoiled DNA. Science. 1992 Aug 21;257(5073):1110–1115. doi: 10.1126/science.257.5073.1110. [DOI] [PubMed] [Google Scholar]
- Schlick T., Olson W. K., Westcott T., Greenberg J. P. On higher buckling transitions in supercoiled DNA. Biopolymers. 1994 May;34(5):565–597. doi: 10.1002/bip.360340502. [DOI] [PubMed] [Google Scholar]
- Shaw S. Y., Wang J. C. Knotting of a DNA chain during ring closure. Science. 1993 Apr 23;260(5107):533–536. doi: 10.1126/science.8475384. [DOI] [PubMed] [Google Scholar]
- Song L., Fujimoto B. S., Wu P. G., Thomas J. C., Shibata J. H., Schurr J. M. Evidence for allosteric transitions in secondary structure induced by superhelical stress. J Mol Biol. 1990 Jul 5;214(1):307–326. doi: 10.1016/0022-2836(90)90163-g. [DOI] [PubMed] [Google Scholar]
- Spengler S. J., Stasiak A., Cozzarelli N. R. The stereostructure of knots and catenanes produced by phage lambda integrative recombination: implications for mechanism and DNA structure. Cell. 1985 Aug;42(1):325–334. doi: 10.1016/s0092-8674(85)80128-8. [DOI] [PubMed] [Google Scholar]
- Stigter D. Interactions of highly charged colloidal cylinders with applications to double-stranded. Biopolymers. 1977 Jul;16(7):1435–1448. doi: 10.1002/bip.1977.360160705. [DOI] [PubMed] [Google Scholar]
- Tan R. K., Harvey S. C. Molecular mechanics model of supercoiled DNA. J Mol Biol. 1989 Feb 5;205(3):573–591. doi: 10.1016/0022-2836(89)90227-1. [DOI] [PubMed] [Google Scholar]
- Taylor W. H., Hagerman P. J. Application of the method of phage T4 DNA ligase-catalyzed ring-closure to the study of DNA structure. II. NaCl-dependence of DNA flexibility and helical repeat. J Mol Biol. 1990 Mar 20;212(2):363–376. doi: 10.1016/0022-2836(90)90131-5. [DOI] [PubMed] [Google Scholar]
- Tesi MC, Janse van Rensburg EJ, Orlandini E, Sumners DW, Whittington SG. Knotting and supercoiling in circular DNA: A model incorporating the effect of added salt. Phys Rev E Stat Phys Plasmas Fluids Relat Interdiscip Topics. 1994 Jan;49(1):868–872. doi: 10.1103/physreve.49.868. [DOI] [PubMed] [Google Scholar]
- Timsit Y., Moras D. Groove-backbone interaction in B-DNA. Implication for DNA condensation and recombination. J Mol Biol. 1991 Oct 5;221(3):919–940. doi: 10.1016/0022-2836(91)80184-v. [DOI] [PubMed] [Google Scholar]
- Timsit Y., Vilbois E., Moras D. Base-pairing shift in the major groove of (CA)n tracts by B-DNA crystal structures. Nature. 1991 Nov 14;354(6349):167–170. doi: 10.1038/354167a0. [DOI] [PubMed] [Google Scholar]
- Timsit Y., Westhof E., Fuchs R. P., Moras D. Unusual helical packing in crystals of DNA bearing a mutation hot spot. Nature. 1989 Oct 5;341(6241):459–462. doi: 10.1038/341459a0. [DOI] [PubMed] [Google Scholar]
- Tsuru H., Wadati M. Elastic model of highly supercoiled DNA. Biopolymers. 1986 Nov;25(11):2083–2096. doi: 10.1002/bip.360251105. [DOI] [PubMed] [Google Scholar]
- Vologodskii A. V., Anshelevich V. V., Lukashin A. V., Frank-Kamenetskii M. D. Statistical mechanics of supercoils and the torsional stiffness of the DNA double helix. Nature. 1979 Jul 26;280(5720):294–298. doi: 10.1038/280294a0. [DOI] [PubMed] [Google Scholar]
- Vologodskii A. V., Cozzarelli N. R. Conformational and thermodynamic properties of supercoiled DNA. Annu Rev Biophys Biomol Struct. 1994;23:609–643. doi: 10.1146/annurev.bb.23.060194.003141. [DOI] [PubMed] [Google Scholar]
- Vologodskii A. V., Levene S. D., Klenin K. V., Frank-Kamenetskii M., Cozzarelli N. R. Conformational and thermodynamic properties of supercoiled DNA. J Mol Biol. 1992 Oct 20;227(4):1224–1243. doi: 10.1016/0022-2836(92)90533-p. [DOI] [PubMed] [Google Scholar]
- Wang J. C. Variation of the average rotation angle of the DNA helix and the superhelical turns of covalently closed cyclic lambda DNA. J Mol Biol. 1969 Jul 14;43(1):25–39. doi: 10.1016/0022-2836(69)90076-x. [DOI] [PubMed] [Google Scholar]