Abstract
1. Electrophysiological techniques are described which allow intracellular recording from peripheral myelinated axons of lizards and frogs for up to several hours. The sciatic and intramuscular axons studied here have resting potentials of -60 to -80 mV and action potentials (evoked by stimulation of the proximal nerve trunk) of 50-90 mV. They show a prominent depolarizing afterpotential (d.a.p.), which is present both in isolated axons and in axons still attached to their peripheral terminals. This d.a.p. has a peak amplitude of 5-20 mV at the resting potential, and decays with a half-time of 20-100 msec.
2. The peak amplitude of the d.a.p. is voltage-sensitive, increasing to up to 26 mV with membrane hyperpolarization. The d.a.p. disappears as the axon is depolarized to -60 to -45 mV, and does not appear to reverse with further depolarization.
3. The d.a.p. is not reduced when bath Ca is replaced by 2-10 mm divalent Mn or Ni. The d.a.p. is not reversed when axons depleted of Cl (by prolonged exposure to Cl-deficient, SO4-enriched solutions) are bathed in Cl-rich solutions. These results suggest that the d.a.p. is not mediated by a conductance change specific for Ca or Cl ions. Partial substitution of tetramethylammonium for bath Na, or addition of 10-5 m-tetrodotoxin to the normal bathing solution, reduces the amplitude of both the action potential and the d.a.p.
4. The amplitude of the d.a.p. is not sensitive to bath [K] over the range 1-7·5 mm, provided that all measurements are made at the same holding potential. This result argues that the d.a.p. is not mediated by accumulation of K outside the active axon.
5. Treatments expected to inhibit the Na—K exchange pump (cooling from 25 to 10 °C, or 0·15 mm-ouabain) do not enlarge or prolong the d.a.p., although they do abolish a slower hyperpolarizing afterpotential seen following repetitive stimulation.
6. The passive voltage response of the axon to small injected pulses of depolarizing or hyperpolarizing current shows a prominent, slowly decaying component with a time course similar to that of the d.a.p. Depolarizing current reduces the input resistance of the axon, and increases the rate of decay of both the passive voltage response and the d.a.p. There is a slight conductance increase during the peak of the d.a.p., but the same conductance increase can be produced by a comparable passive depolarization.
7. We conclude that the d.a.p. is due mainly to a passive capacitative current, probably resulting from discharge of the internodal axonal membrane capacitance through a resistive current pathway beneath or through the myelin sheath. We suggest that this slow capacitative discharge becomes evident as soon as most of the nodal ionic channels activated during the action potential have closed. An electrical model of the myelinated axon that incorporates the postulated internodal leakage pathway can account both for the prolonged d.a.p. recorded inside the axon, and for the potential profile recorded extra-axonally in or near the internodal periaxonal space.
Full text
PDF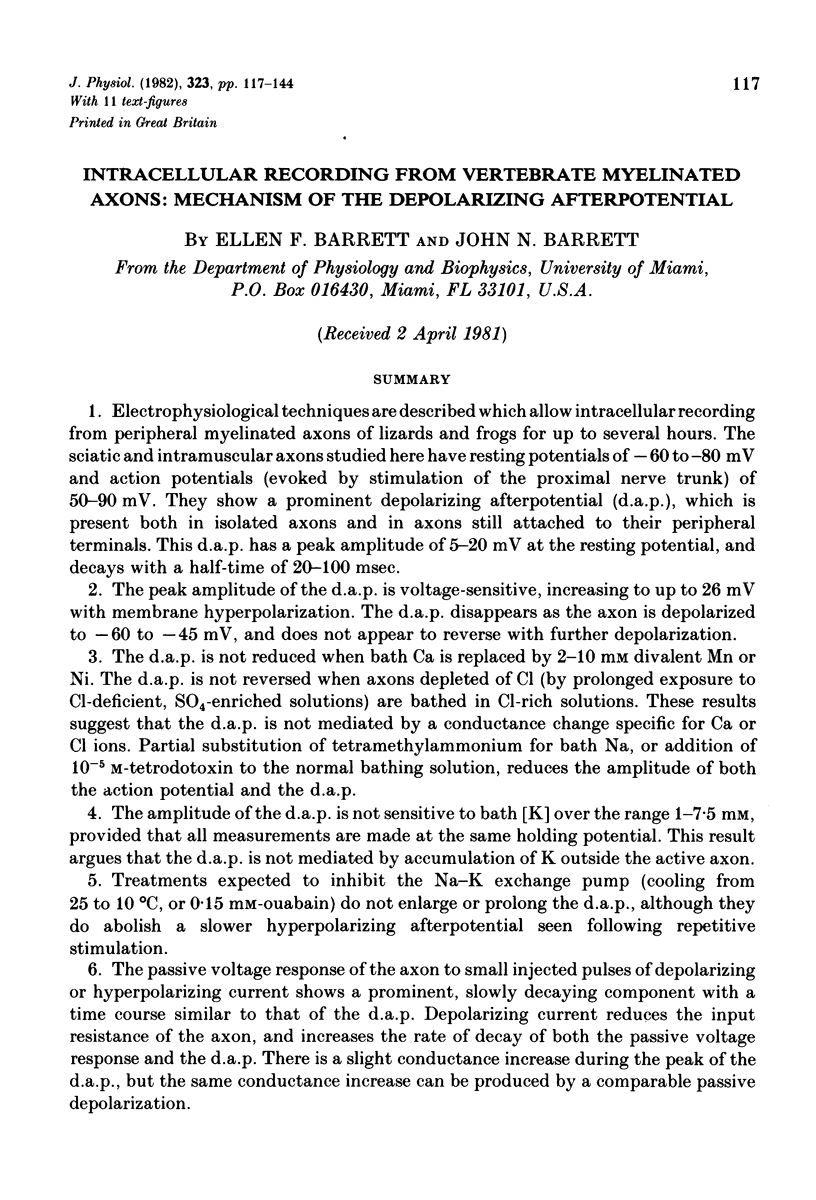
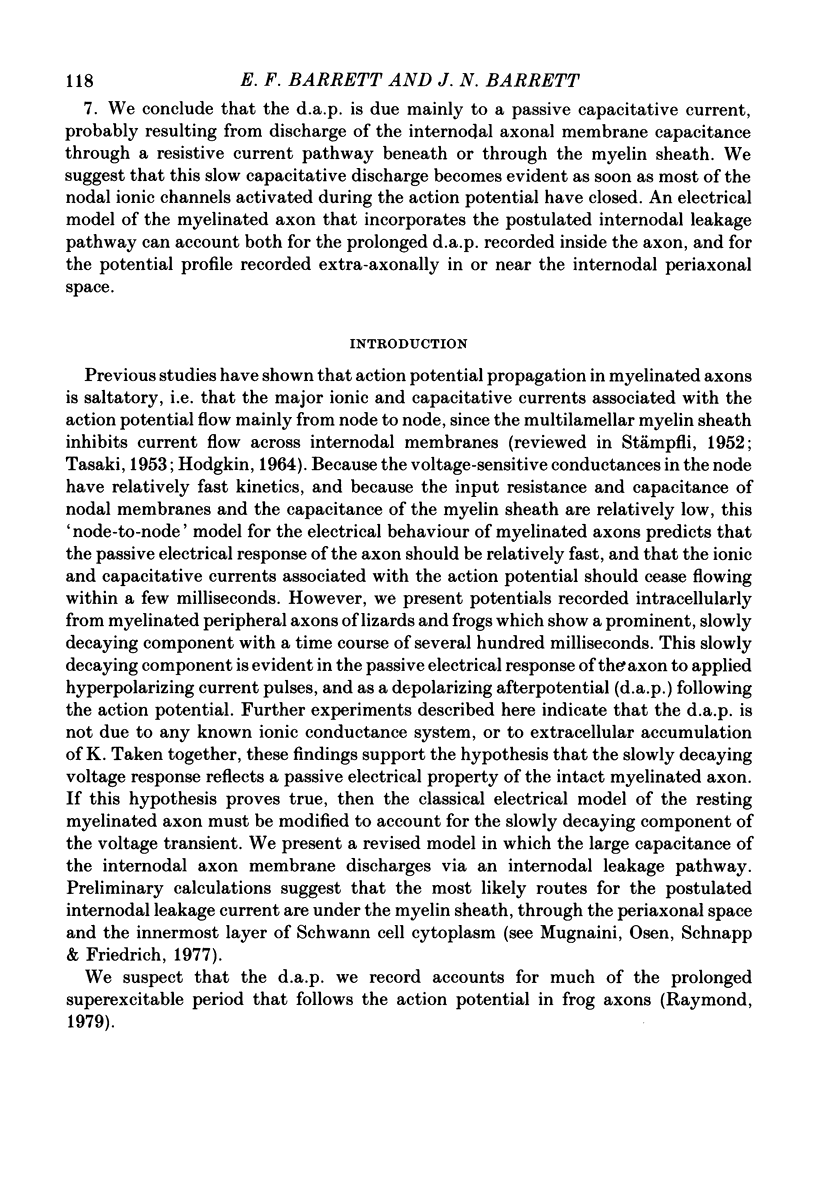
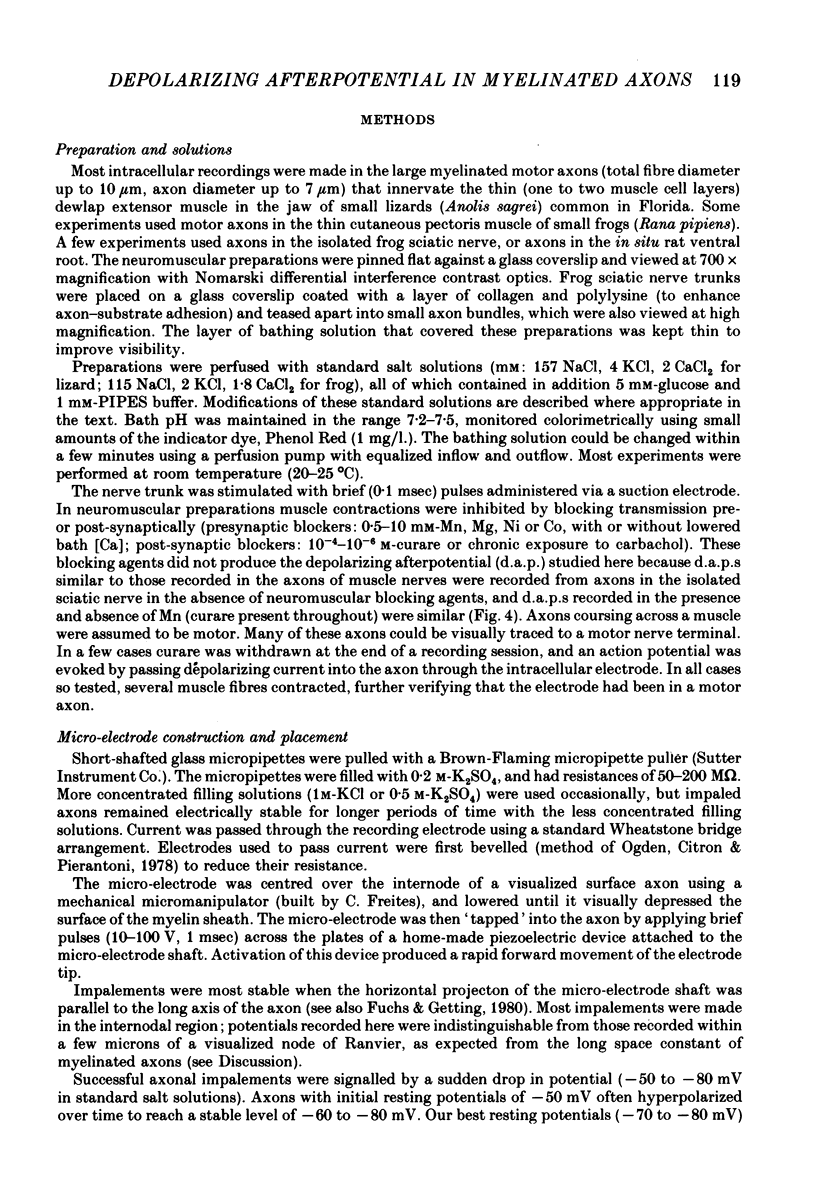
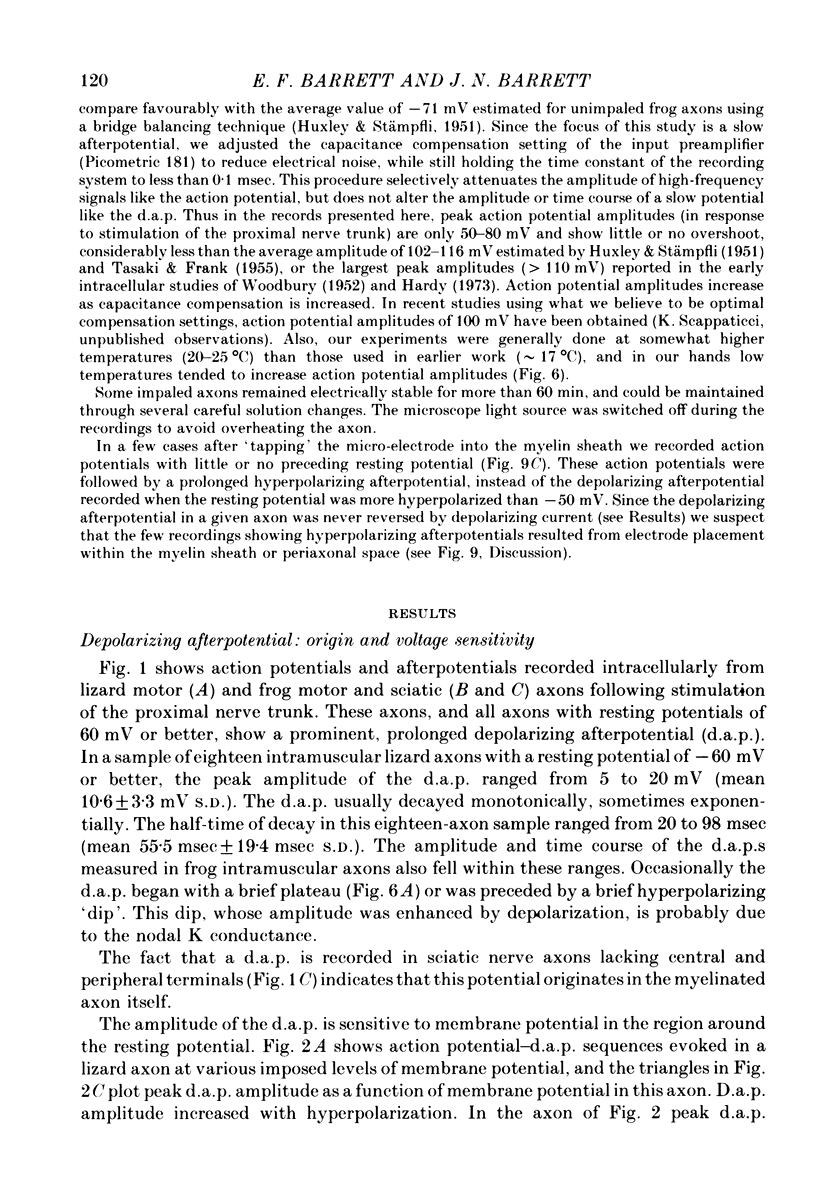
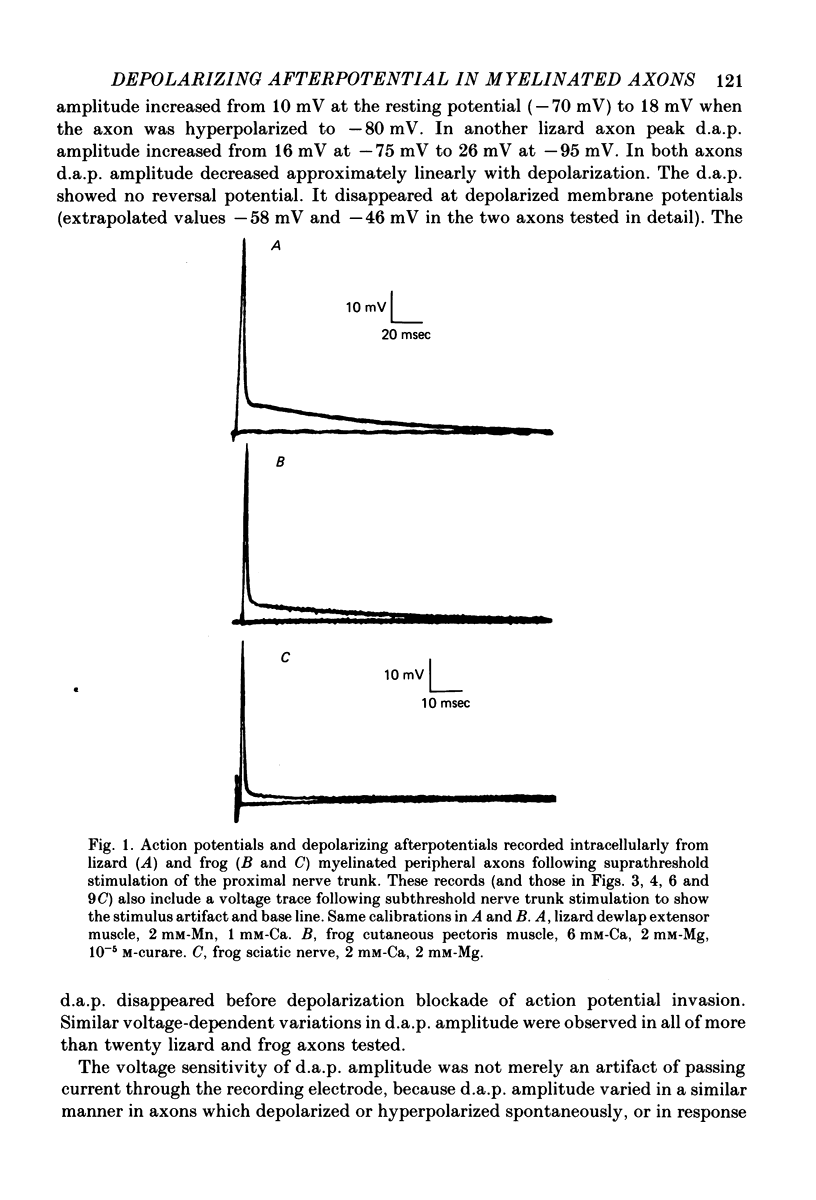
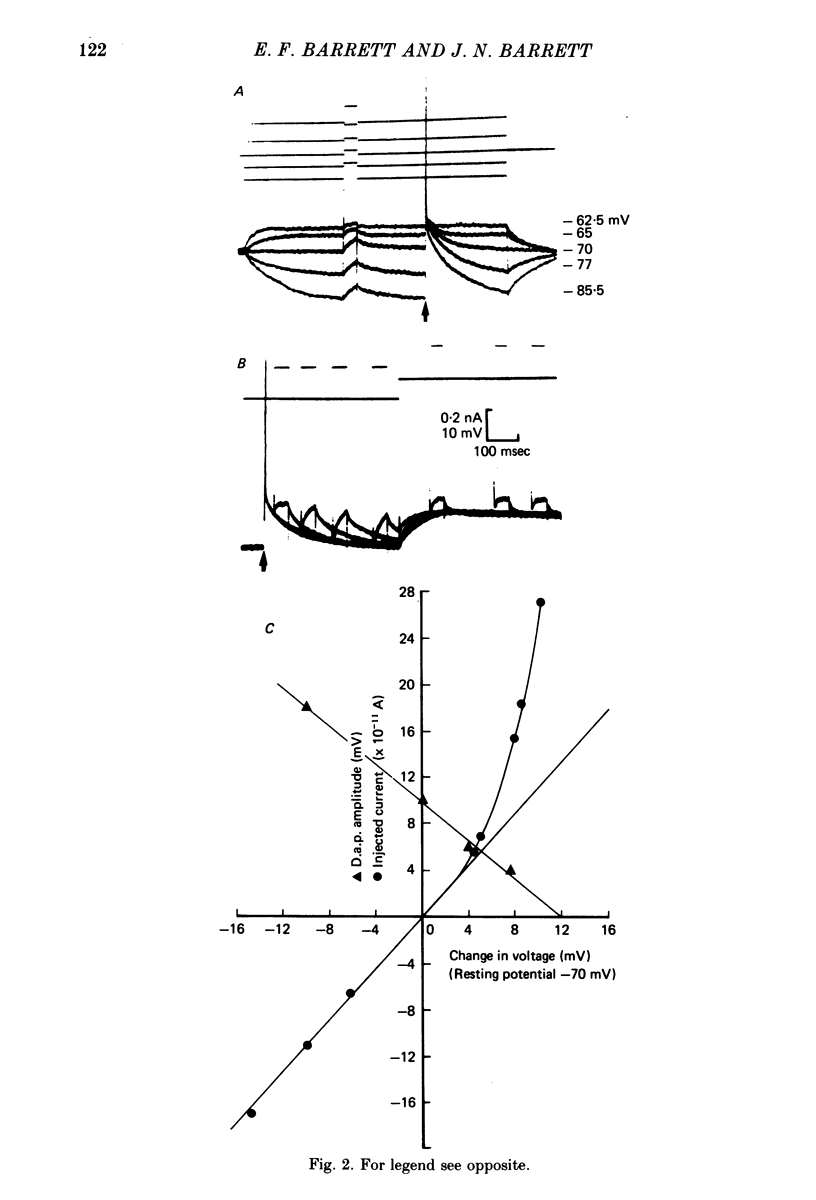
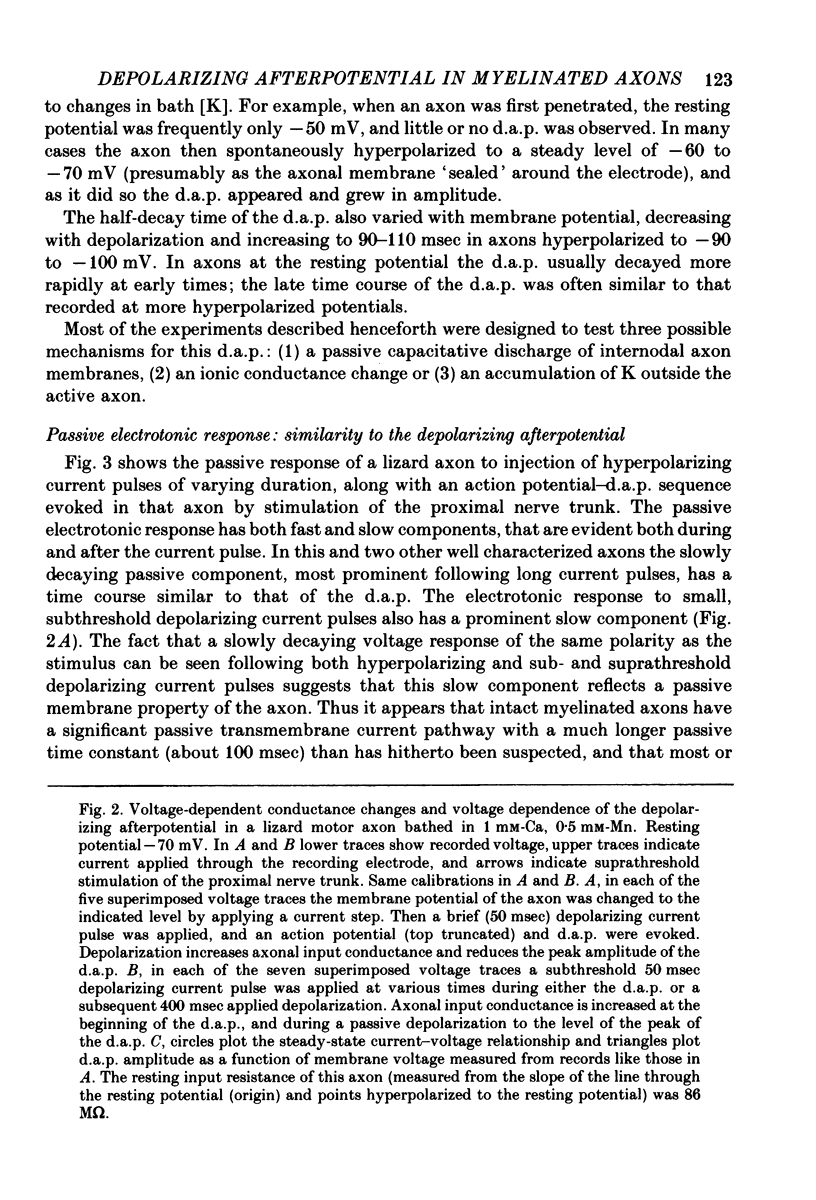
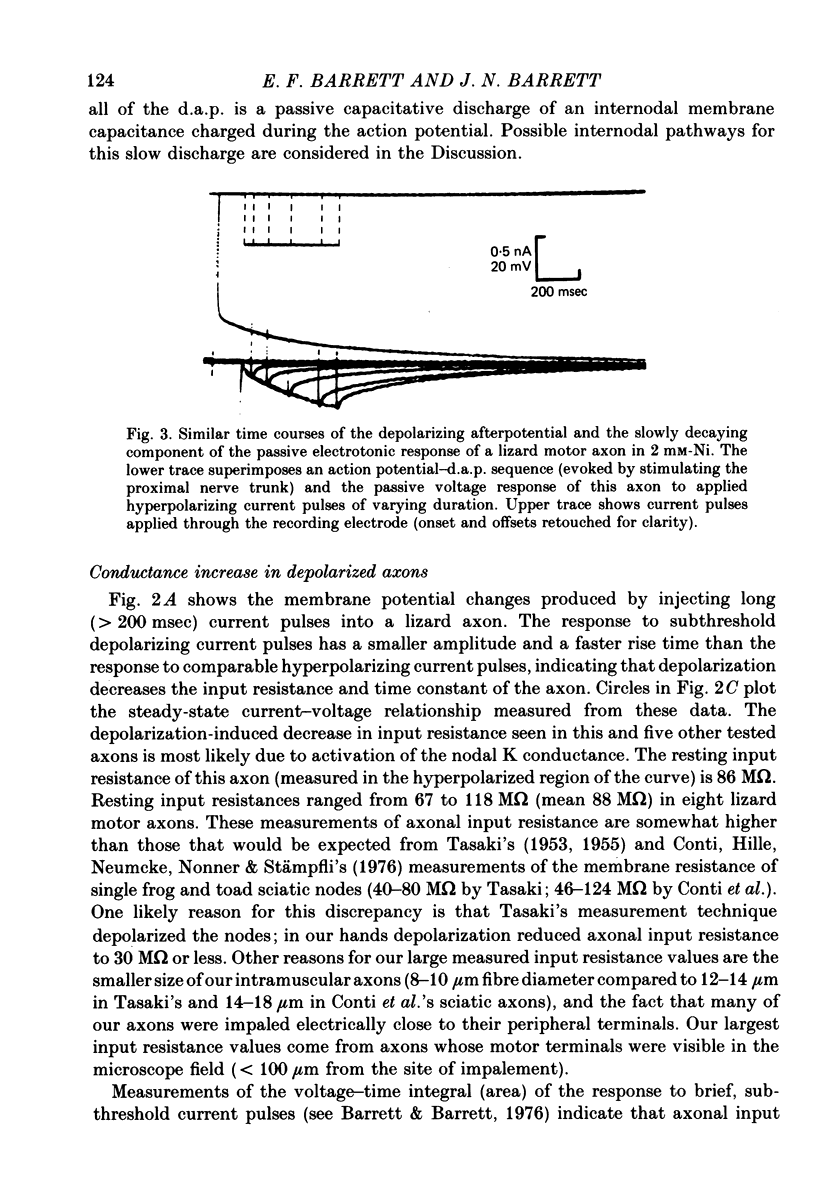
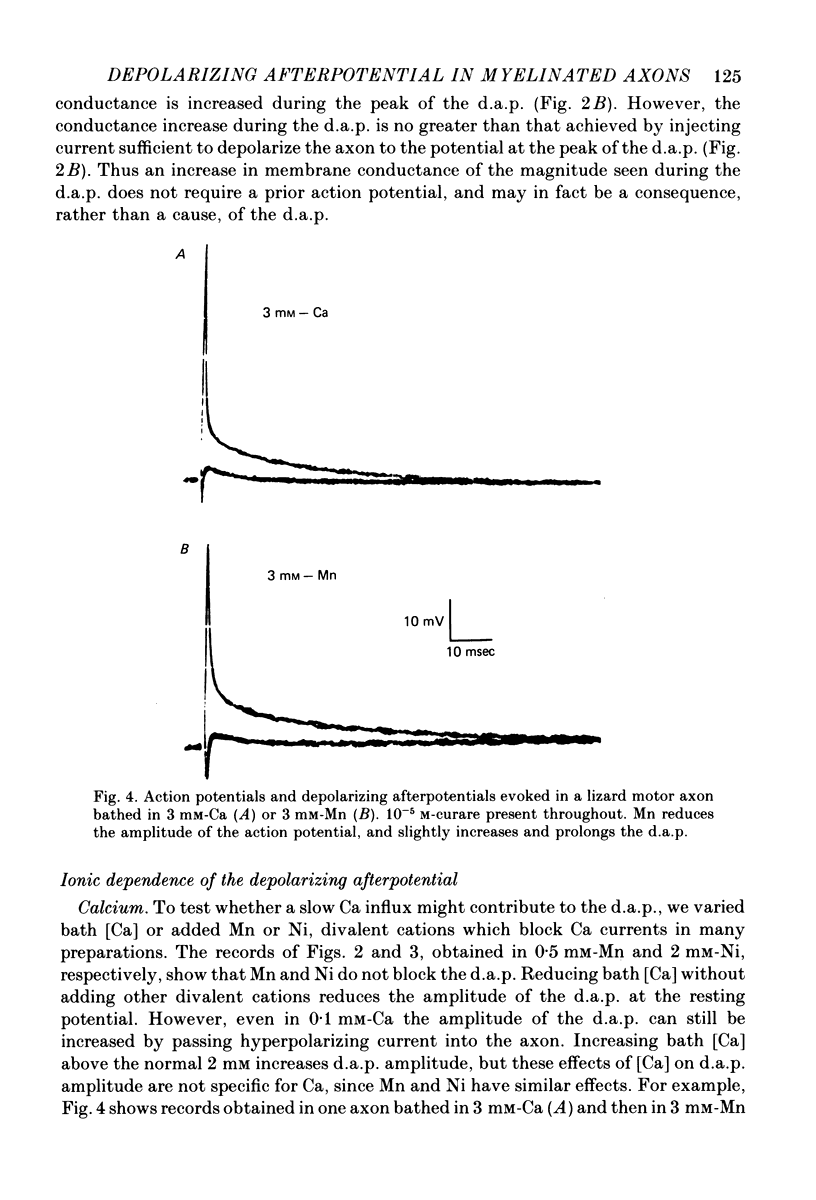
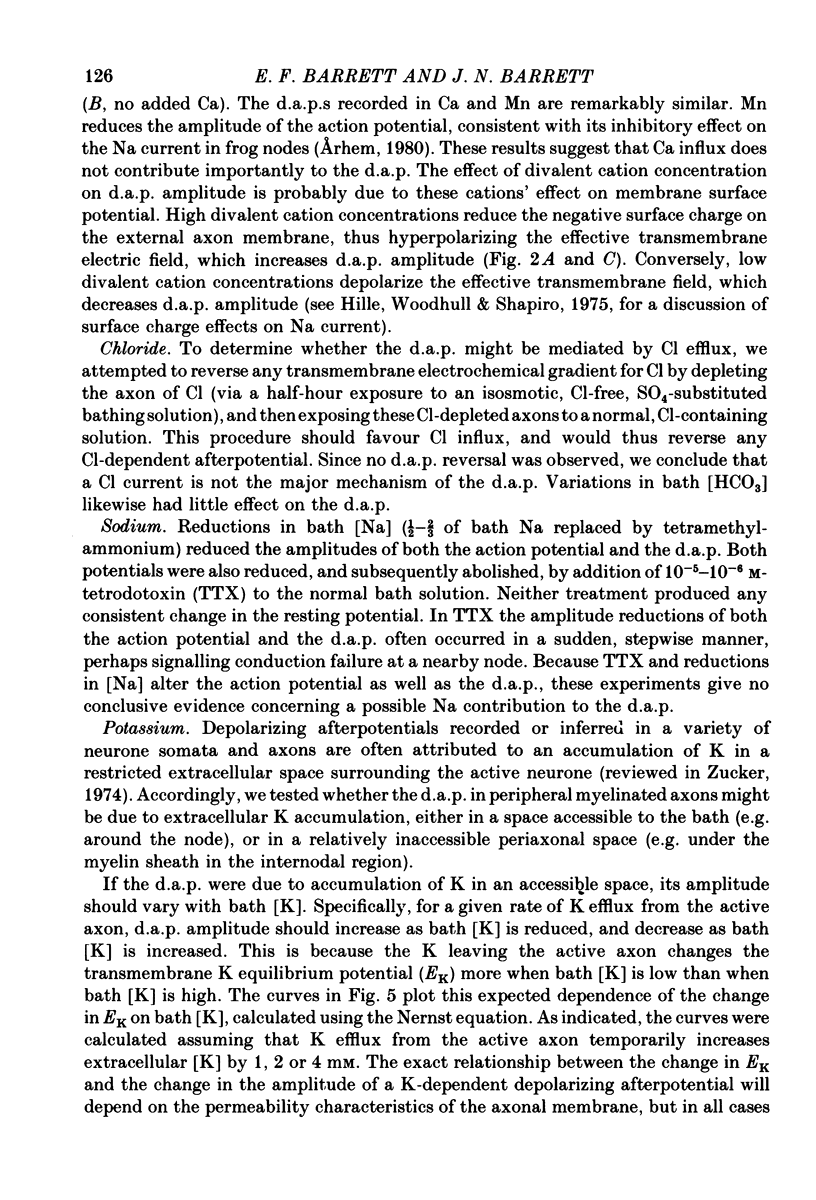
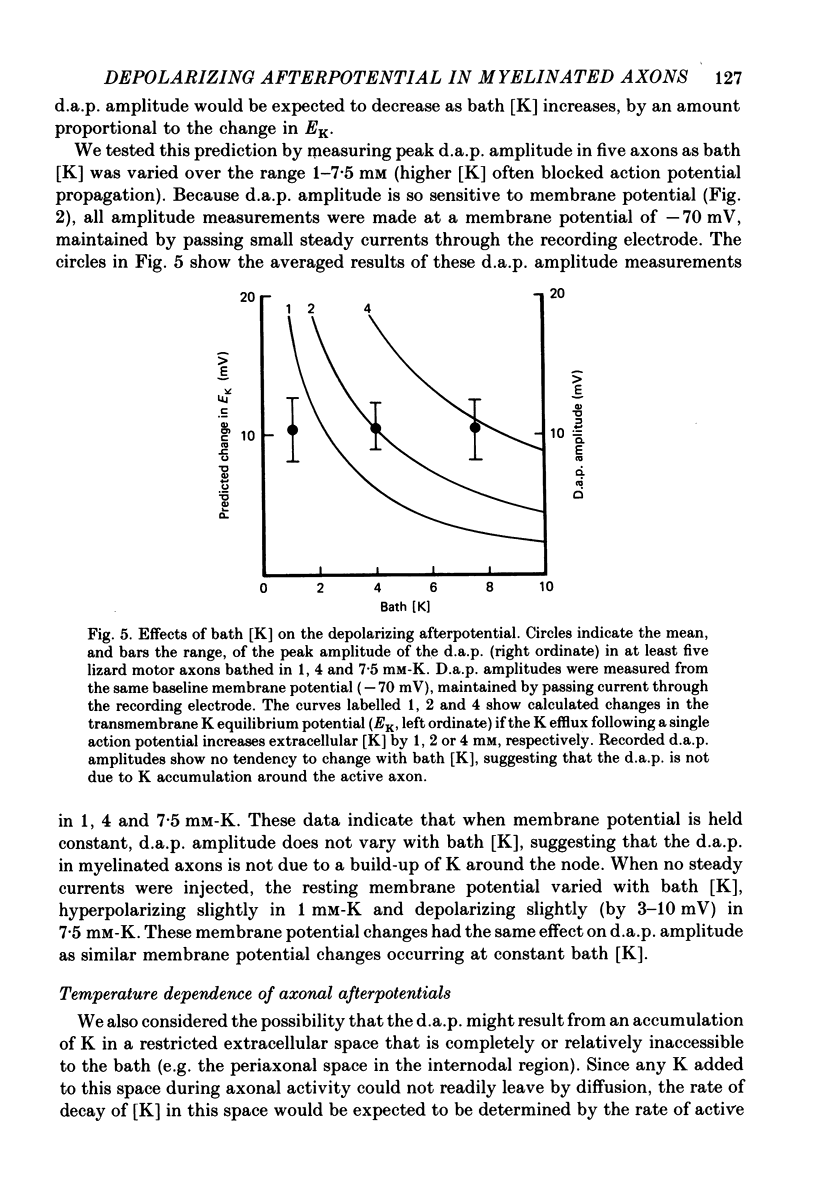
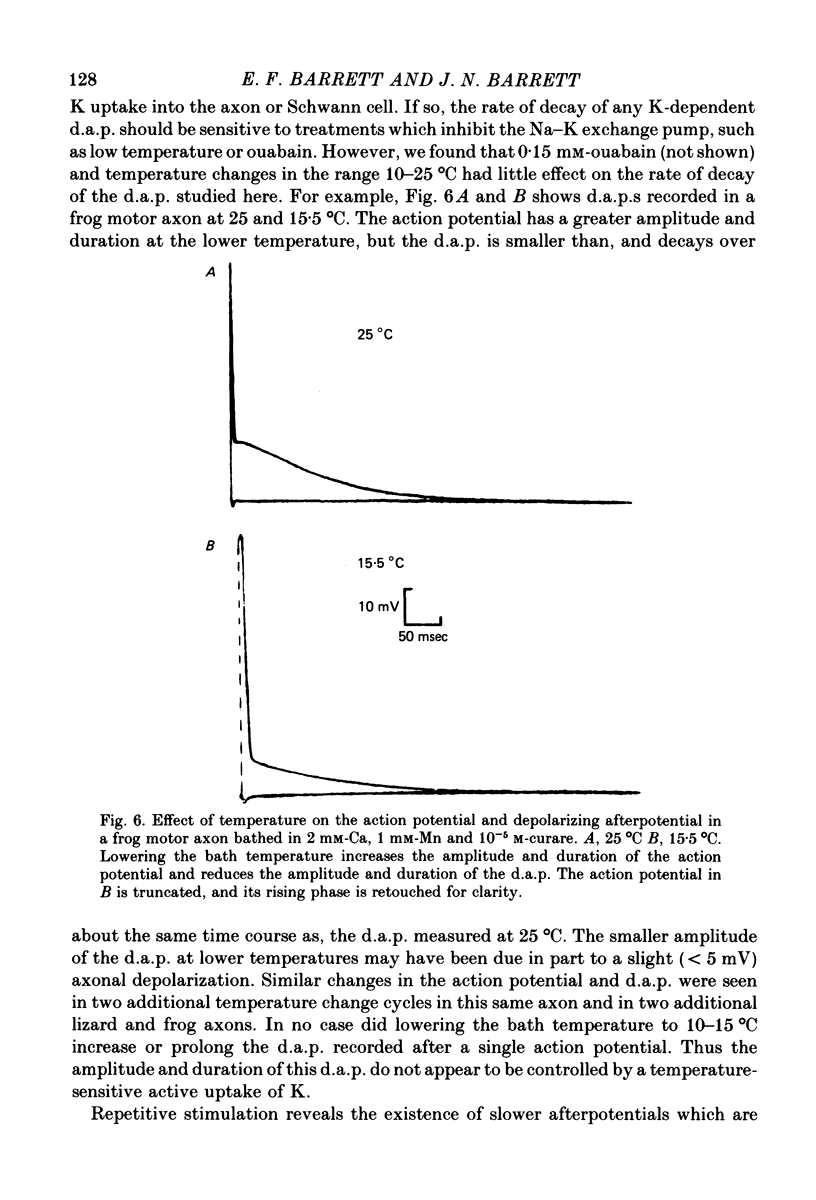
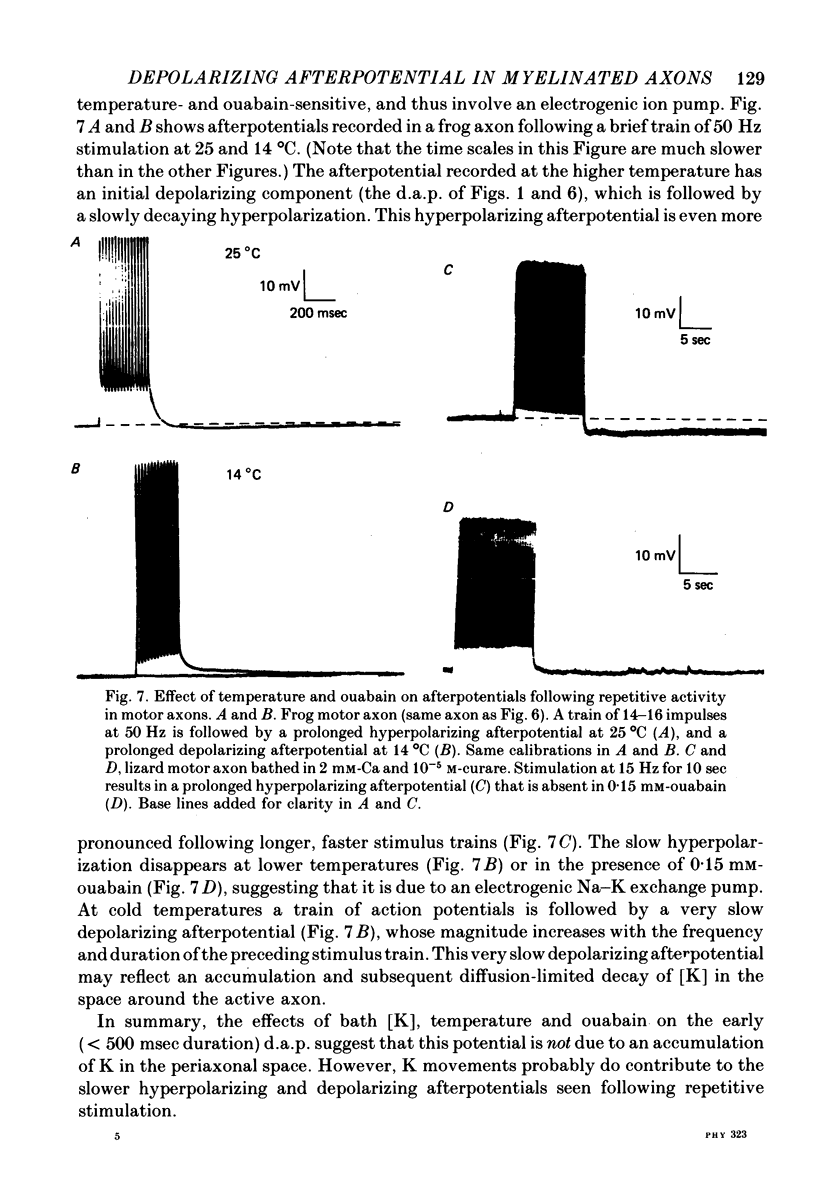
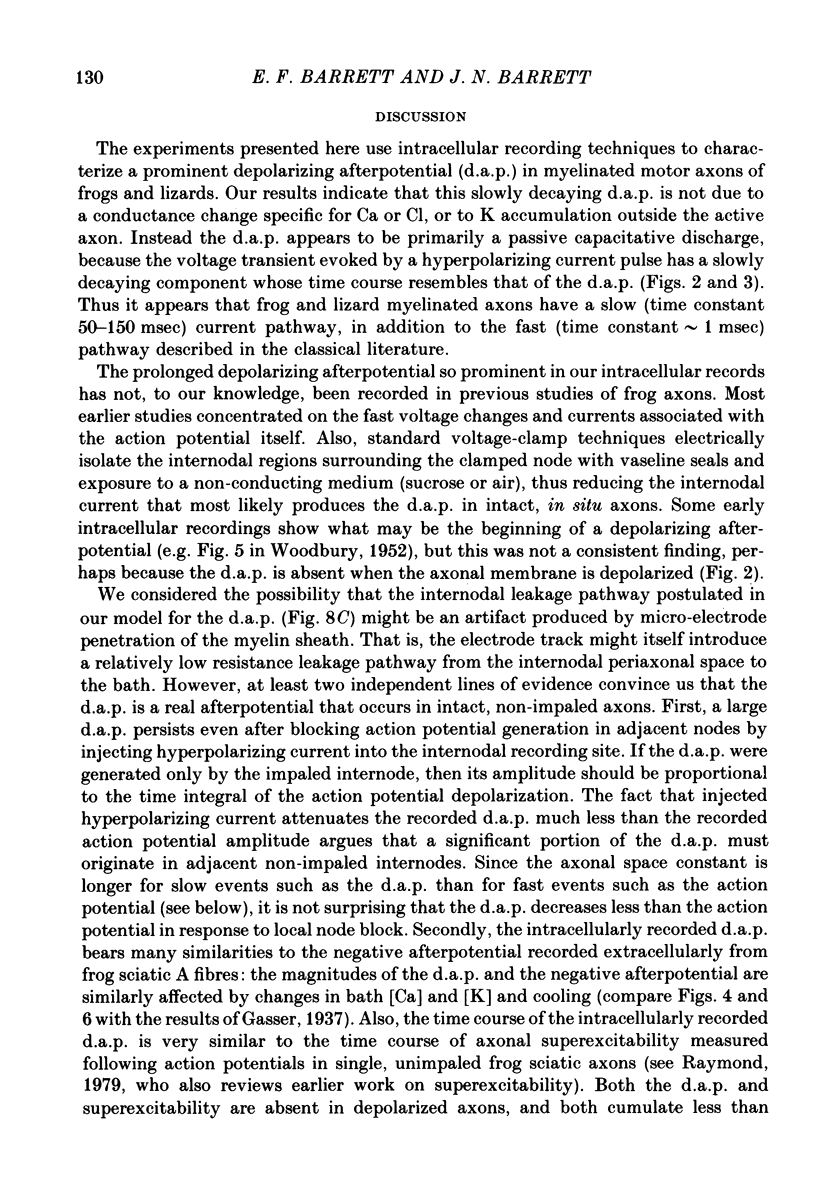
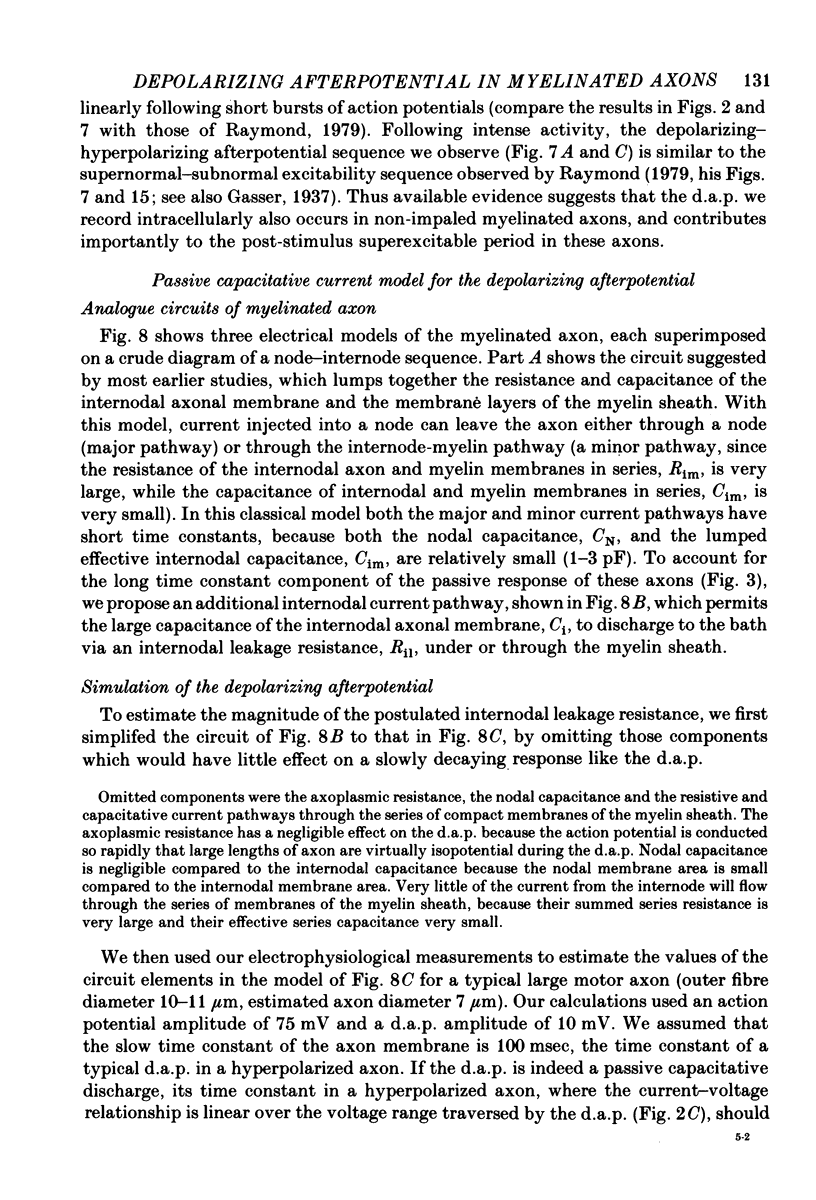
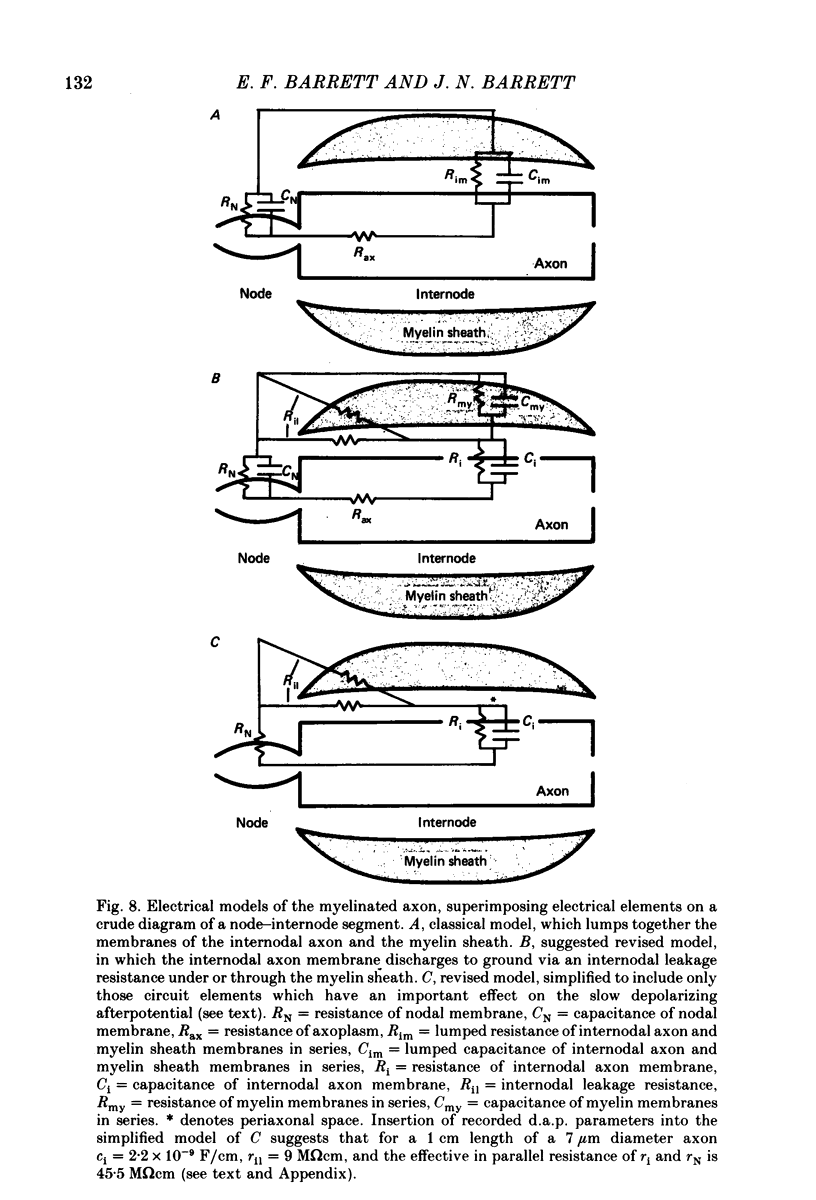
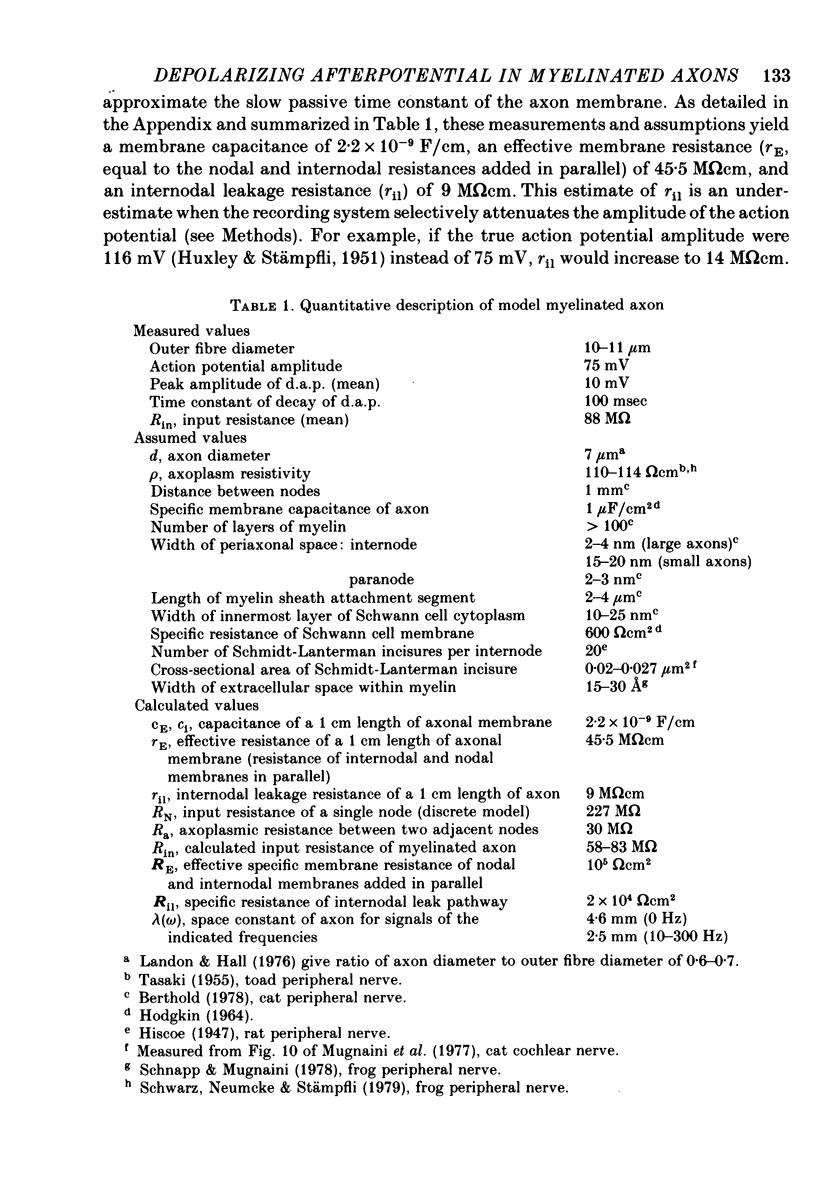
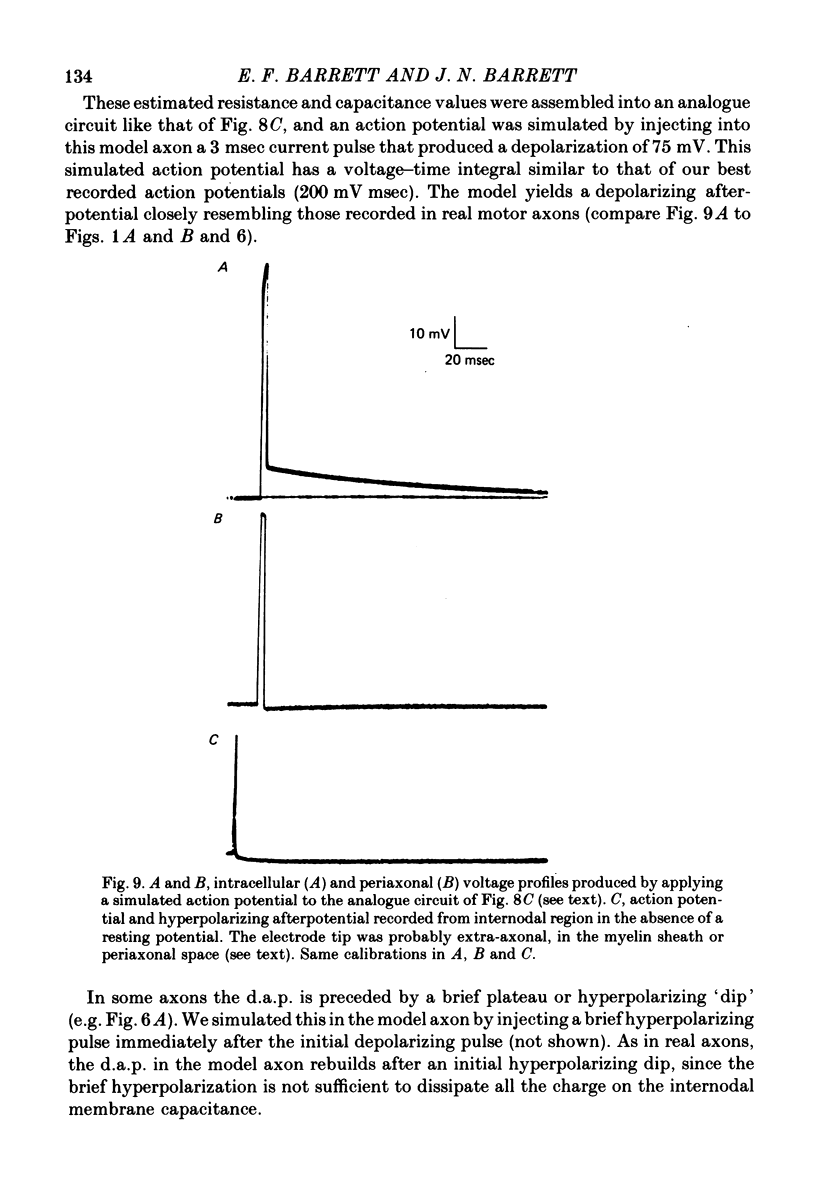
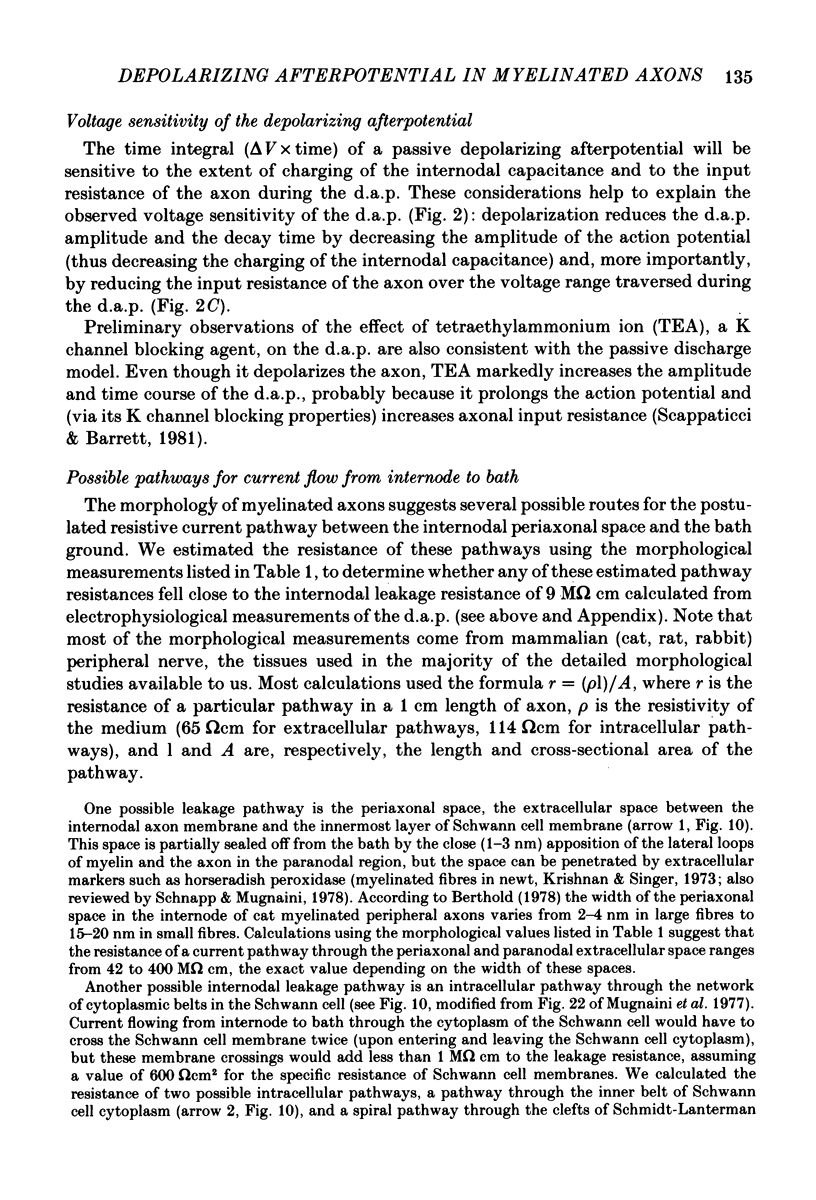
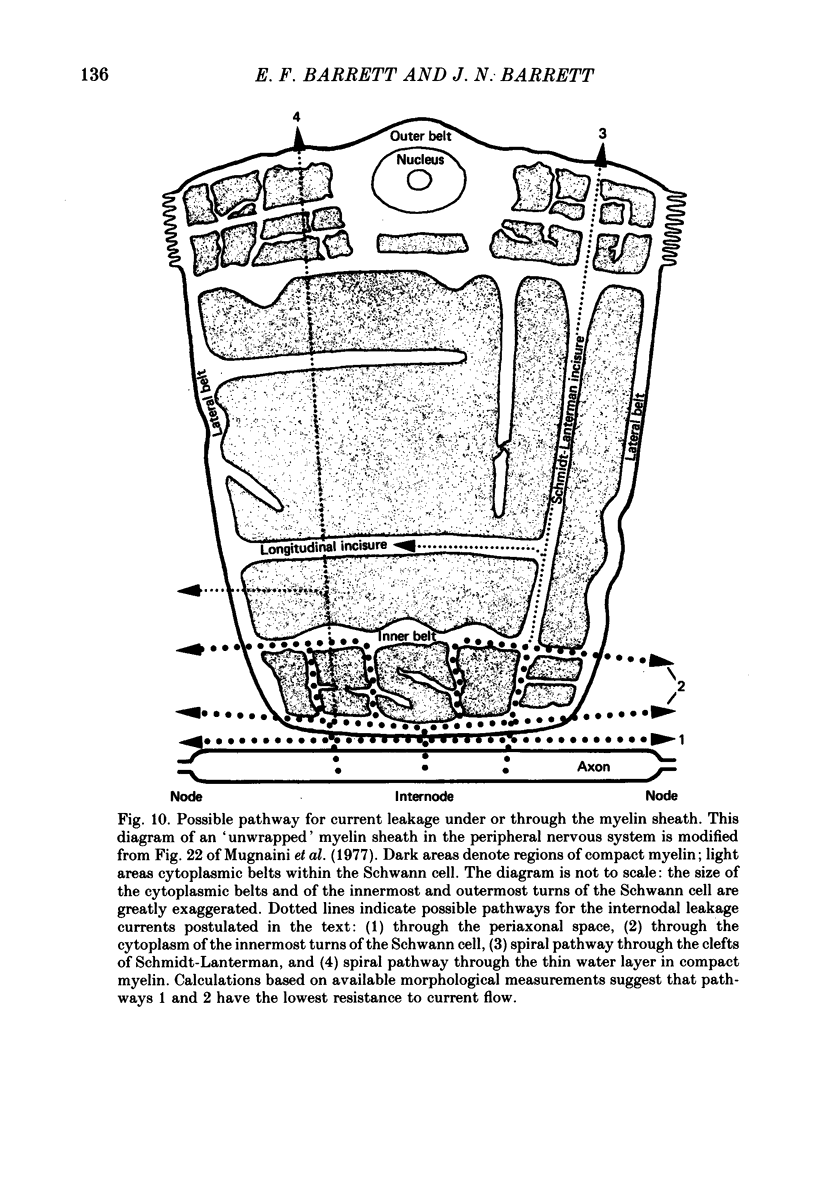
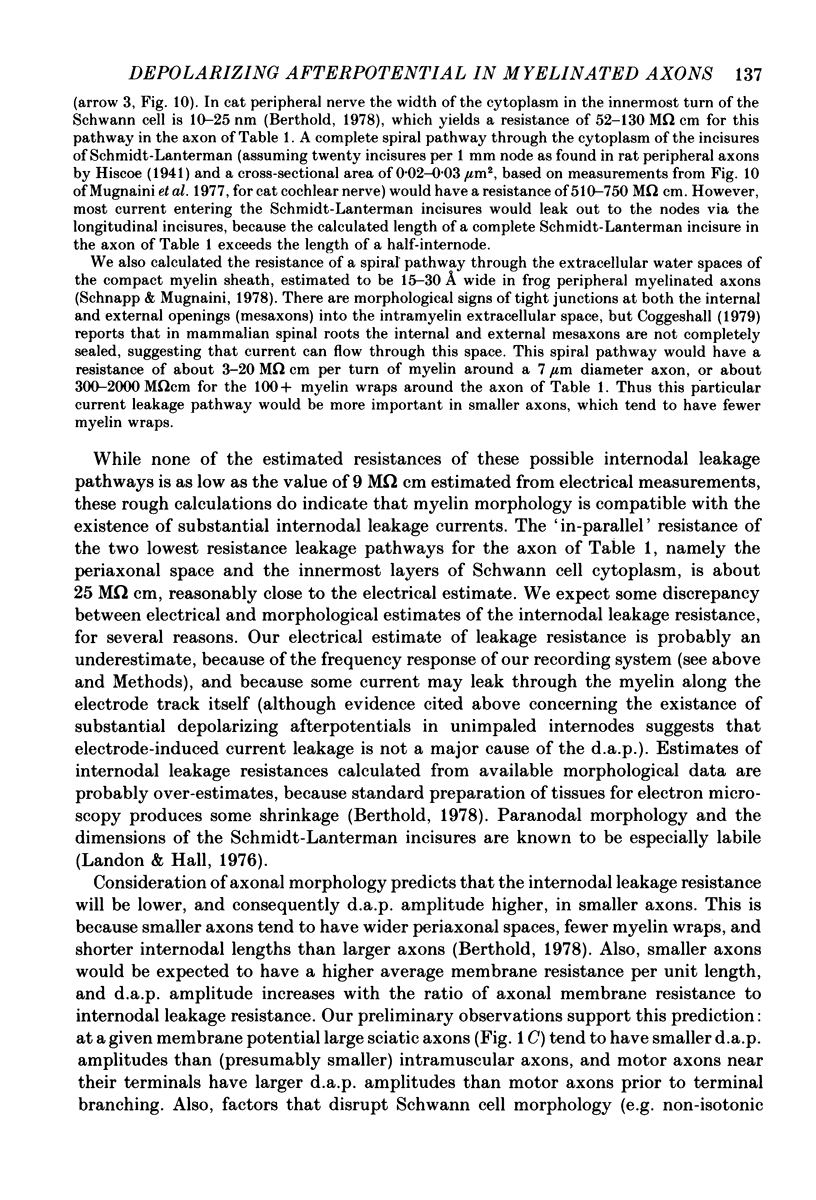
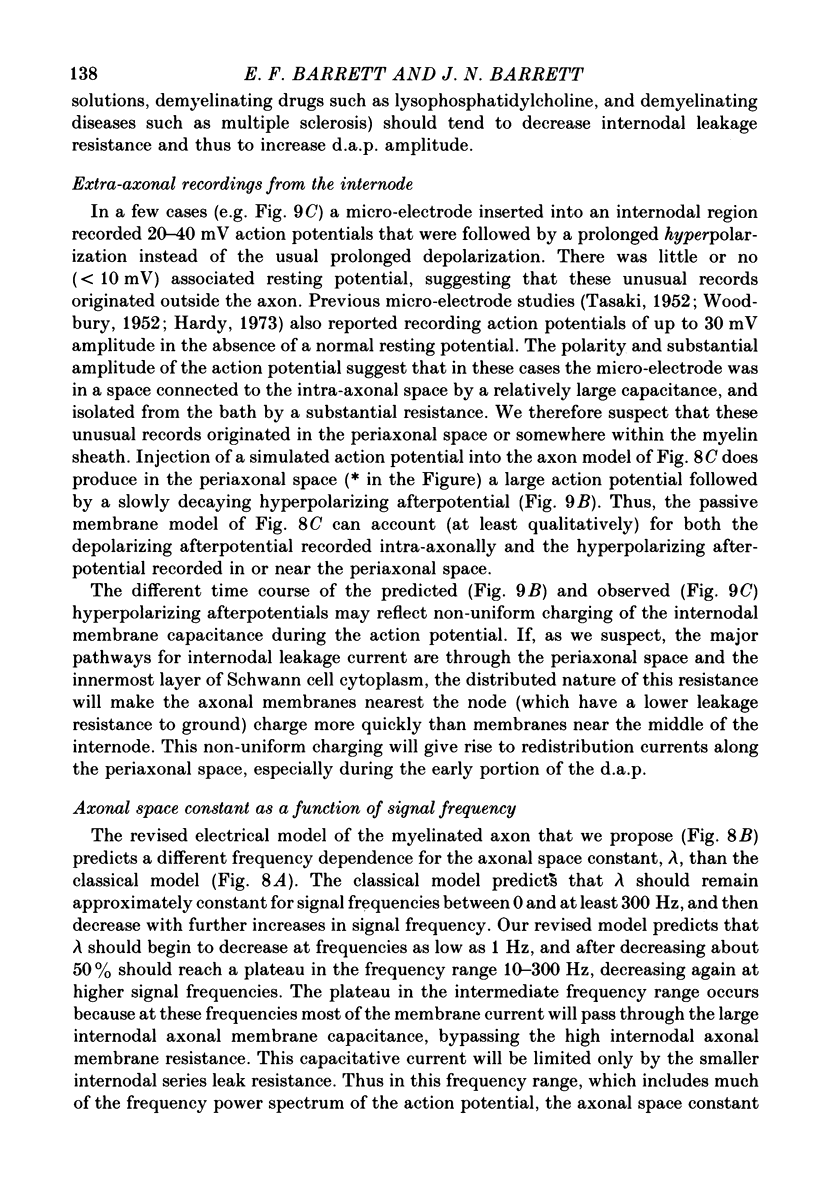
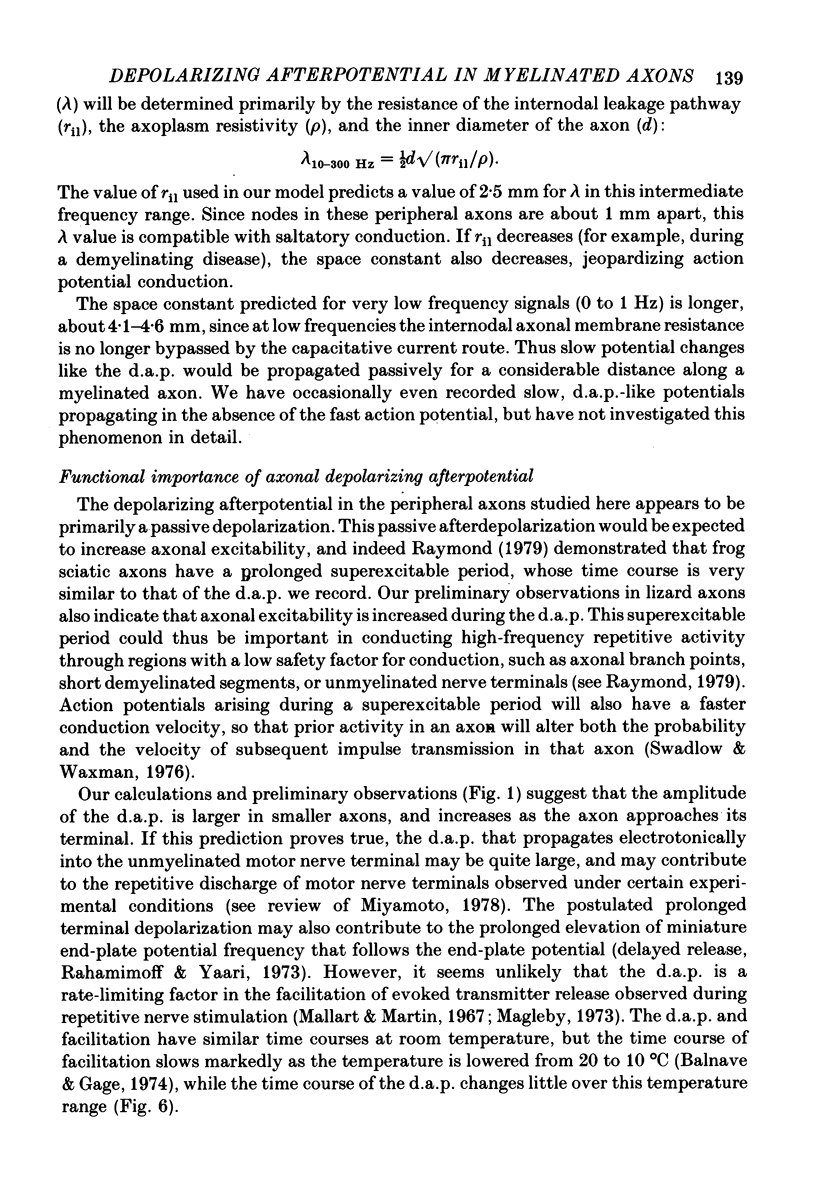
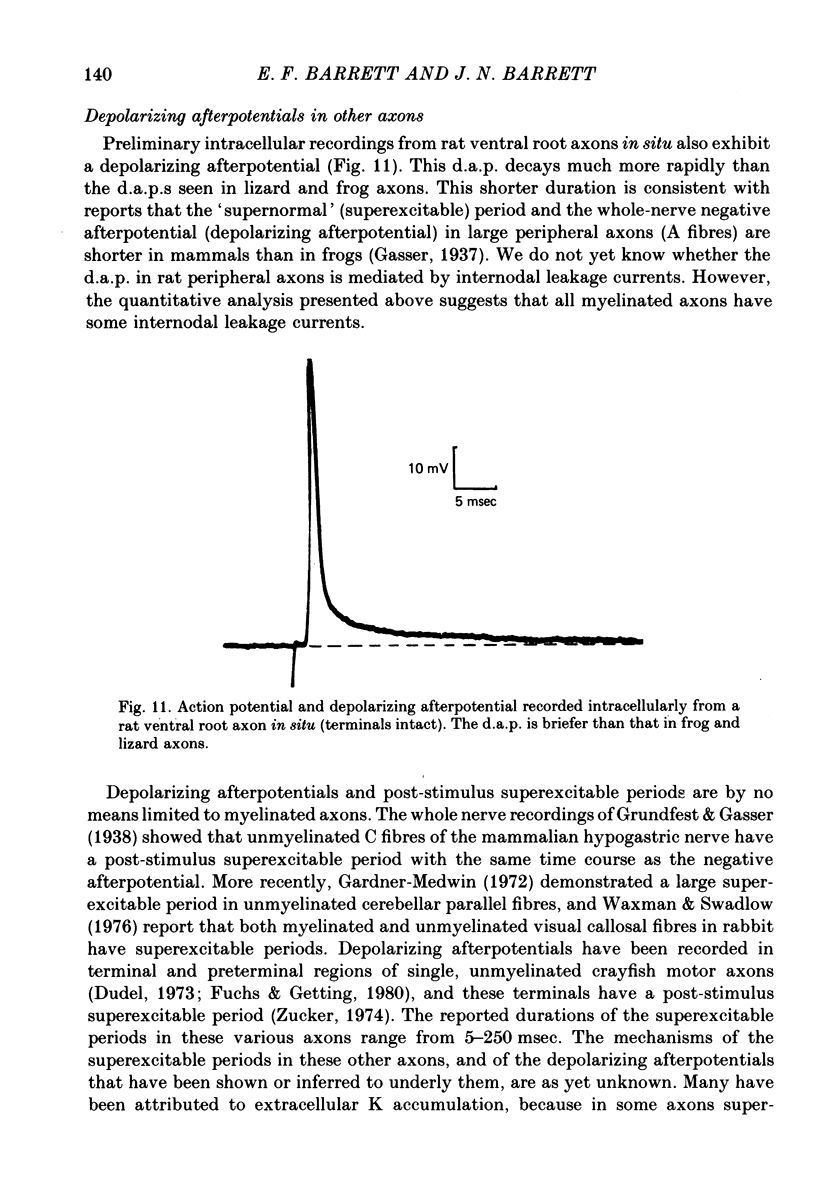
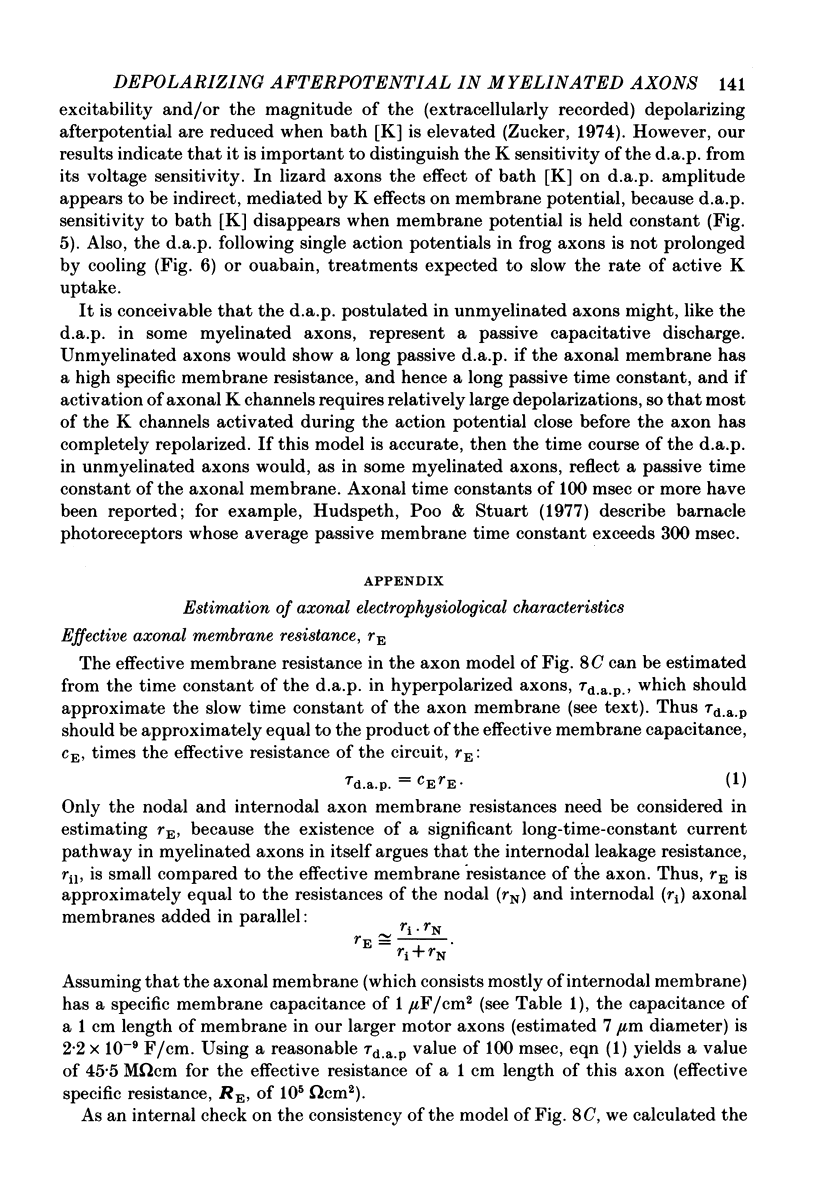
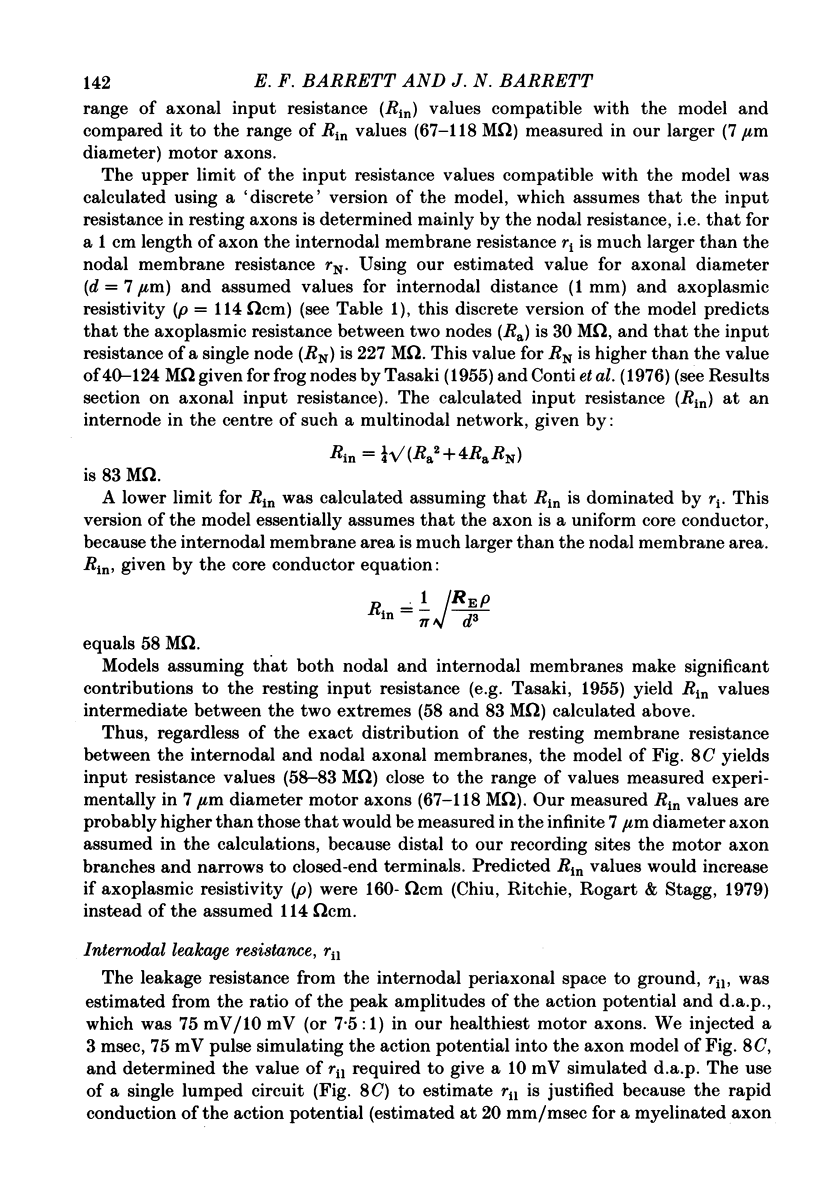
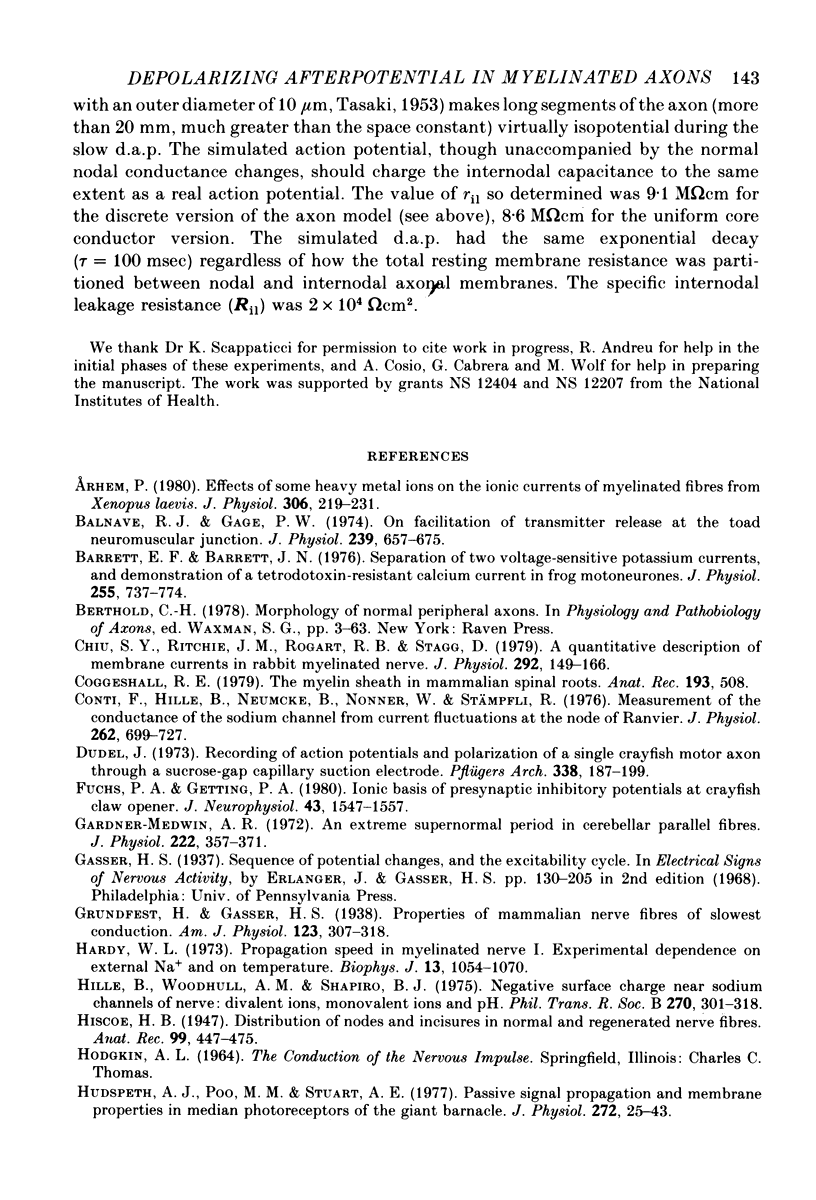
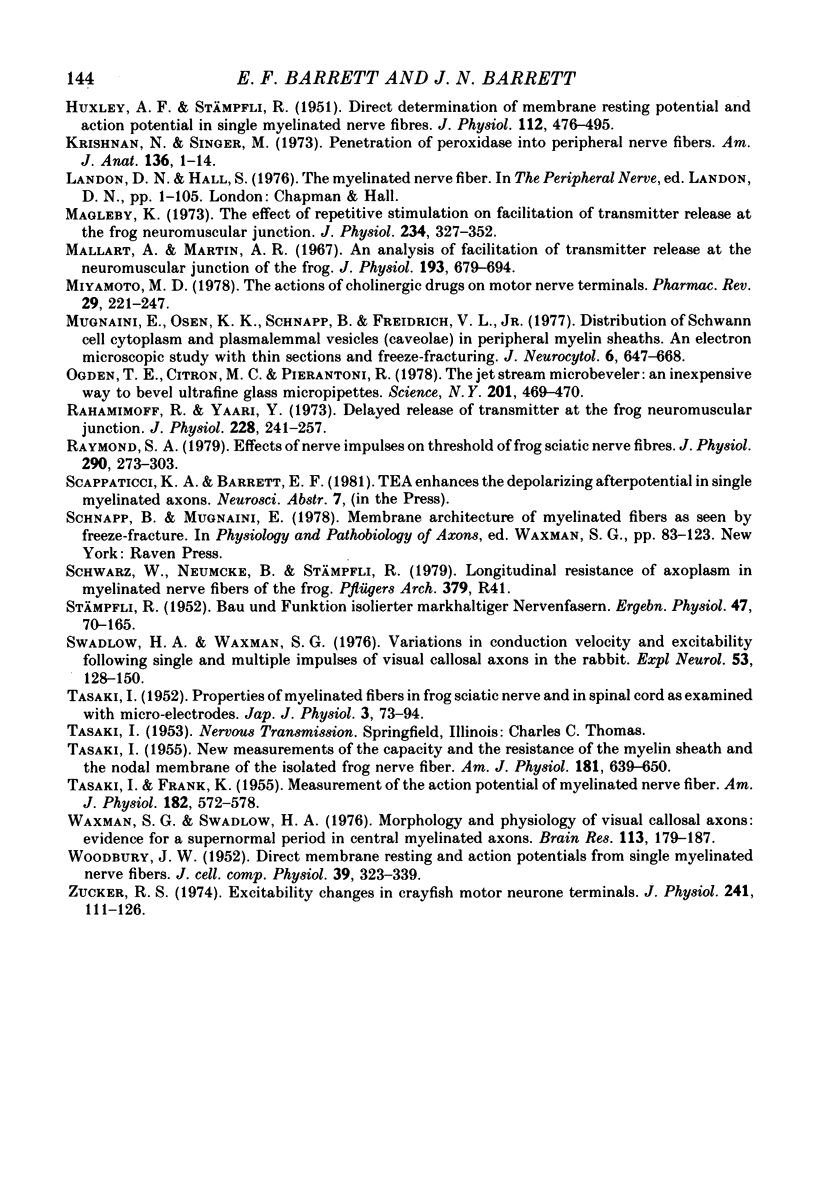
Selected References
These references are in PubMed. This may not be the complete list of references from this article.
- Arhem P. Effects of some heavy metal ions on the ionic currents of myelinated fibres from Xenopus laevis. J Physiol. 1980 Sep;306:219–231. doi: 10.1113/jphysiol.1980.sp013393. [DOI] [PMC free article] [PubMed] [Google Scholar]
- Balnave R. J., Gage P. W. On facilitation of transmitter release at the toad neuromuscular junction. J Physiol. 1974 Jun;239(3):657–675. doi: 10.1113/jphysiol.1974.sp010588. [DOI] [PMC free article] [PubMed] [Google Scholar]
- Barrett E. F., Barret J. N. Separation of two voltage-sensitive potassium currents, and demonstration of a tetrodotoxin-resistant calcium current in frog motoneurones. J Physiol. 1976 Mar;255(3):737–774. doi: 10.1113/jphysiol.1976.sp011306. [DOI] [PMC free article] [PubMed] [Google Scholar]
- Chiu S. Y., Ritchie J. M., Rogart R. B., Stagg D. A quantitative description of membrane currents in rabbit myelinated nerve. J Physiol. 1979 Jul;292:149–166. doi: 10.1113/jphysiol.1979.sp012843. [DOI] [PMC free article] [PubMed] [Google Scholar]
- Conti F., Hille B., Neumcke B., Nonner W., Stämpfli R. Measurement of the conductance of the sodium channel from current fluctuations at the node of Ranvier. J Physiol. 1976 Nov;262(3):699–727. doi: 10.1113/jphysiol.1976.sp011616. [DOI] [PMC free article] [PubMed] [Google Scholar]
- Dudel J. Recording of action potentials and polarization of a single crayfish motor axon through a sucrose-gap capillary suction electrode. Pflugers Arch. 1973 Feb 6;338(3):187–199. doi: 10.1007/BF00587386. [DOI] [PubMed] [Google Scholar]
- Fuchs P. A., Getting P. A. Ionic basis of presynaptic inhibitory potentials at crayfish claw opener. J Neurophysiol. 1980 Jun;43(6):1547–1557. doi: 10.1152/jn.1980.43.6.1547. [DOI] [PubMed] [Google Scholar]
- Gardner-Medwin A. R. An extreme supernormal period in cerebellar parallel fibres. J Physiol. 1972 Apr;222(2):357–371. doi: 10.1113/jphysiol.1972.sp009802. [DOI] [PMC free article] [PubMed] [Google Scholar]
- HUXLEY A. F., STAMPFLI R. Direct determination of membrane resting potential and action potential in single myelinated nerve fibers. J Physiol. 1951 Feb;112(3-4):476–495. doi: 10.1113/jphysiol.1951.sp004545. [DOI] [PMC free article] [PubMed] [Google Scholar]
- Hardy W. L. Propagation speed in myelinated nerve. I. Experimental dependence on external Na and on temperature. Biophys J. 1973 Oct;13(10):1054–1070. doi: 10.1016/S0006-3495(73)86045-X. [DOI] [PMC free article] [PubMed] [Google Scholar]
- Hille B., Woodhull A. M., Shapiro B. I. Negative surface charge near sodium channels of nerve: divalent ions, monovalent ions, and pH. Philos Trans R Soc Lond B Biol Sci. 1975 Jun 10;270(908):301–318. doi: 10.1098/rstb.1975.0011. [DOI] [PubMed] [Google Scholar]
- Hudspeth A. J., Poo M. M., Stuart A. E. Passive signal propagation and membrane properties in median photoreceptors of the giant barnacle. J Physiol. 1977 Oct;272(1):25–43. doi: 10.1113/jphysiol.1977.sp012032. [DOI] [PMC free article] [PubMed] [Google Scholar]
- Krishnan N., Singer M. Penetration of peroxidase into peripheral nerve fibers. Am J Anat. 1973 Jan;136(1):1–14. doi: 10.1002/aja.1001360102. [DOI] [PubMed] [Google Scholar]
- Magleby K. L. The effect of repetitive stimulation on facilitation of transmitter release at the frog neuromuscular junction. J Physiol. 1973 Oct;234(2):327–352. doi: 10.1113/jphysiol.1973.sp010348. [DOI] [PMC free article] [PubMed] [Google Scholar]
- Mallart A., Martin A. R. An analysis of facilitation of transmitter release at the neuromuscular junction of the frog. J Physiol. 1967 Dec;193(3):679–694. doi: 10.1113/jphysiol.1967.sp008388. [DOI] [PMC free article] [PubMed] [Google Scholar]
- Miyamoto M. D. The actions of cholinergic drugs on motor nerve terminals. Pharmacol Rev. 1977 Sep;29(3):221–247. [PubMed] [Google Scholar]
- Mugnaini E., Osen K. K., Schnapp B., Friedrich V. L., Jr Distribution of Schwann cell cytoplasm and plasmalemmal vesicles (caveolae) in peripheral myelin sheaths. An electron microscopic study with thin sections and freeze-fracturing. J Neurocytol. 1977 Dec;6(6):647–668. doi: 10.1007/BF01176378. [DOI] [PubMed] [Google Scholar]
- Ogden T. E., Citron M. C., Pierantoni R. The jet stream microbeveler: an inexpensive way to bevel ultrafine glass micropipettes. Science. 1978 Aug 4;201(4354):469–470. doi: 10.1126/science.663670. [DOI] [PubMed] [Google Scholar]
- Rahamimoff R., Yaari Y. Delayed release of transmitter at the frog neuromuscular junction. J Physiol. 1973 Jan;228(1):241–257. doi: 10.1113/jphysiol.1973.sp010084. [DOI] [PMC free article] [PubMed] [Google Scholar]
- Raymond S. A. Effects of nerve impulses on threshold of frog sciatic nerve fibres. J Physiol. 1979 May;290(2):273–303. doi: 10.1113/jphysiol.1979.sp012771. [DOI] [PMC free article] [PubMed] [Google Scholar]
- STAMPFLI R. Bau und Funktion isolierter markhaltiger Nervenfasern. Ergeb Physiol. 1952;47:70–165. [PubMed] [Google Scholar]
- Swadlow H. A., Waxman S. G. Variations in conduction velocity and excitability following single and multiple impulses of visual callosal axons in the rabbit. Exp Neurol. 1976 Oct;53(1):128–150. doi: 10.1016/0014-4886(76)90288-0. [DOI] [PubMed] [Google Scholar]
- TASAKI I., FRANK K. Measurement of the action potential of myelinated nerve fiber. Am J Physiol. 1955 Sep;182(3):572–578. doi: 10.1152/ajplegacy.1955.182.3.572. [DOI] [PubMed] [Google Scholar]
- TASAKI I. New measurements of the capacity and the resistance of the myelin sheath and the nodal membrane of the isolated frog nerve fiber. Am J Physiol. 1955 Jun;181(3):639–650. doi: 10.1152/ajplegacy.1955.181.3.639. [DOI] [PubMed] [Google Scholar]
- TASAKI I. Properties of myelinated fibers in frog sciatic nerve and in spinal cord as examined with micro-electrodes. Jpn J Physiol. 1952 Nov;3(1):73–94. doi: 10.2170/jjphysiol.3.73. [DOI] [PubMed] [Google Scholar]
- WOODBURY J. W. Direct membrane resting and action potentials from single myelinated nerve fibers. J Cell Physiol. 1952 Apr;39(2):323–339. doi: 10.1002/jcp.1030390210. [DOI] [PubMed] [Google Scholar]
- Waxman S. G., Swadlow H. A. Morphology and physiology of visual callosal axons: evidence for a supernormal period in central myelinated axons. Brain Res. 1976 Aug 20;113(1):179–187. doi: 10.1016/0006-8993(76)90017-2. [DOI] [PubMed] [Google Scholar]
- Zucker R. S. Excitability changes in crayfish motor neurone terminals. J Physiol. 1974 Aug;241(1):111–126. doi: 10.1113/jphysiol.1974.sp010643. [DOI] [PMC free article] [PubMed] [Google Scholar]