Abstract
The repulsive pressure between filaments in the lattice of skinned rabbit and frog striated muscle in rigor has been measured as a function of interfilament spacing, using the osmotic pressure generated by solutions of large, uncharged polymeric molecules (dextran and polyvinylpyrrolidone). The pressure/spacing measurements have been compared with theoretically derived curves for electrostatic pressure. In both muscles, the major part of the experimental curves (100-2,000 torr) lies in the same region as the electrostatic pressure curves, providing that a thick filament charge diameter of approximately 30 nm in rabbit and approximately 26 nm in frog is assumed. In chemically skinned or glycerol-extracted rabbit muscle the fit is good; in chemically skinned frog sartorius and semitendinosus muscle the fit is poor, particularly at lower pressures where a greater spacing is observed than expected on theoretical grounds. The charge diameter is much larger than the generally accepted value for thick filament backbone diameter. This may be because electron microscope results have underestimated the amount of filament shrinkage during sample preparation, or because most of the filament charge is located at some distance from the backbone surface, e.g., on HMM-S2. Decreasing the ionic strength of the external solution, changing the pH, and varying the sarcomere length all give pressure/spacing changes similar to those expected from electrostatic pressure calculations. We conclude that over most of the external pressure range studied, repulsive pressure in the lattice is predominantly electrostatic.
Full text
PDF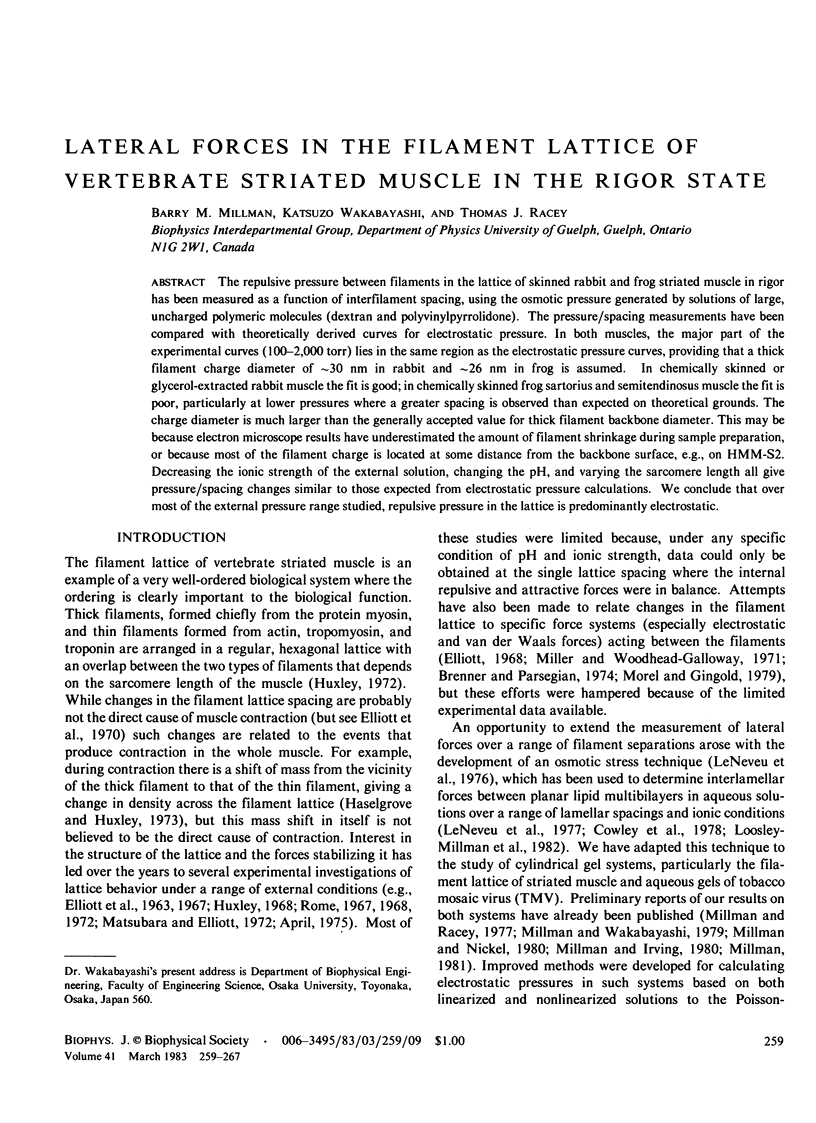
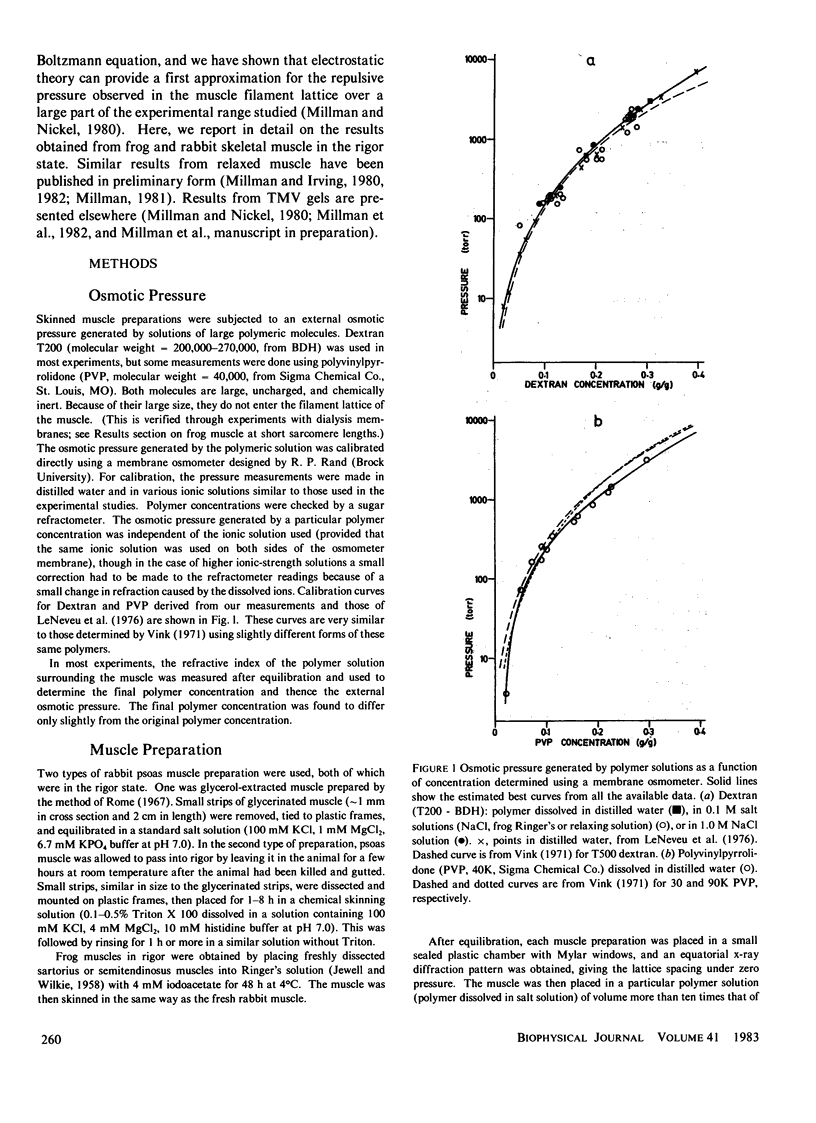
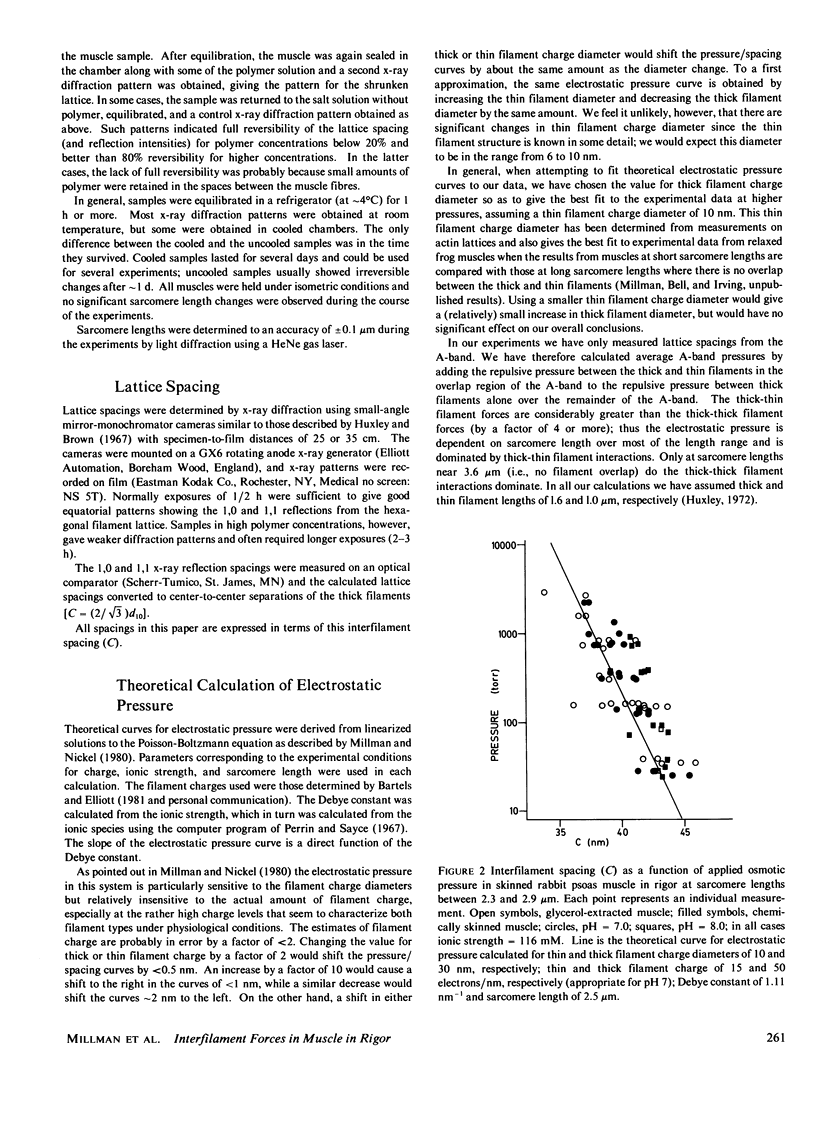
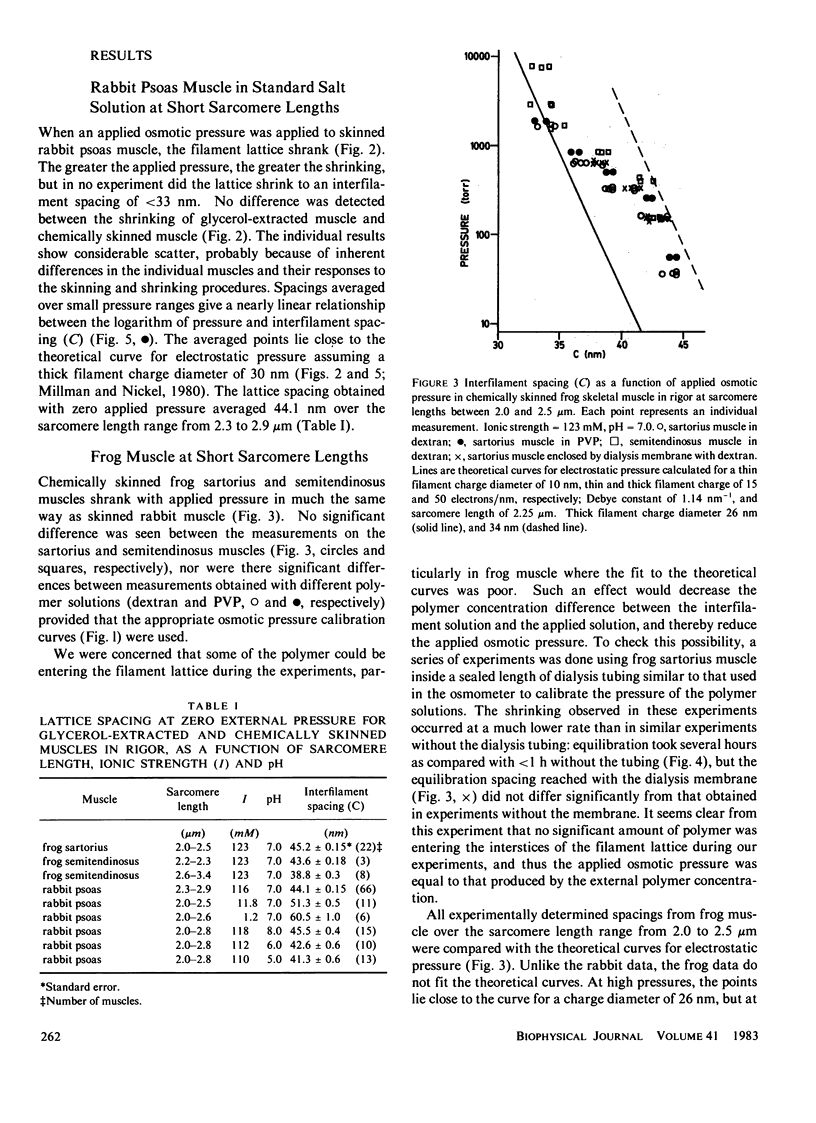
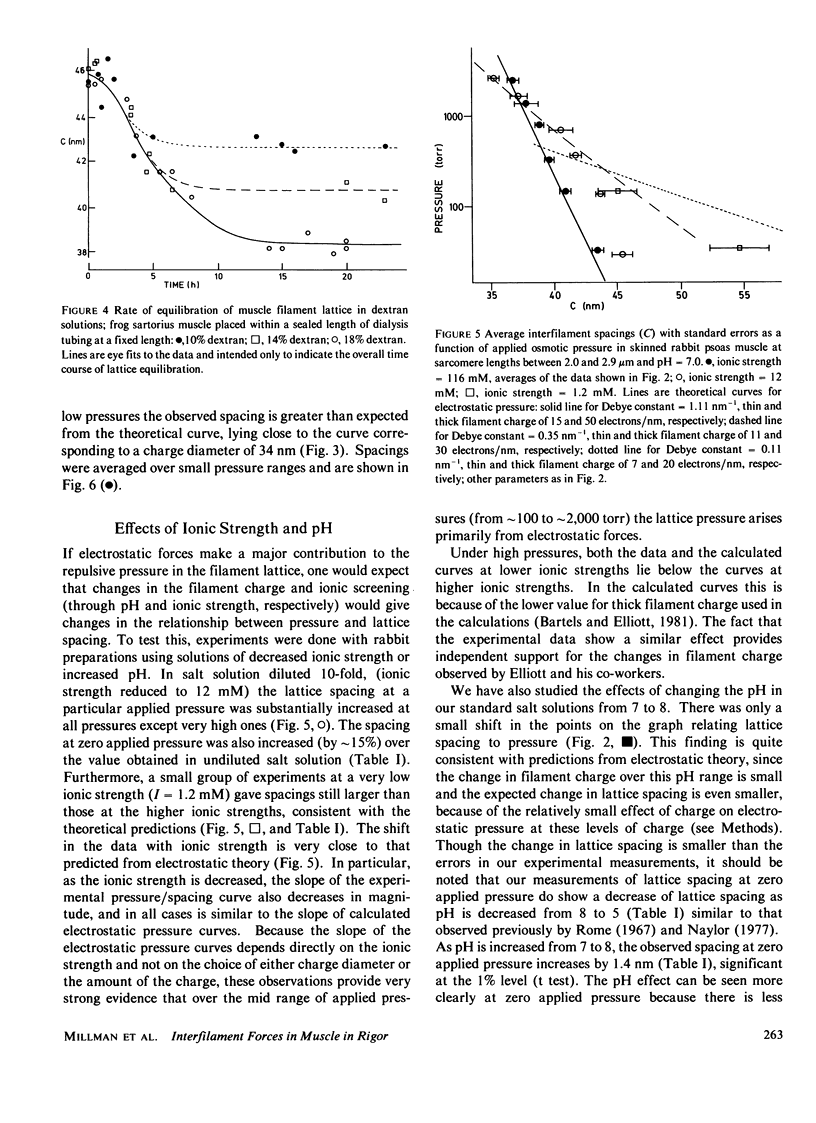
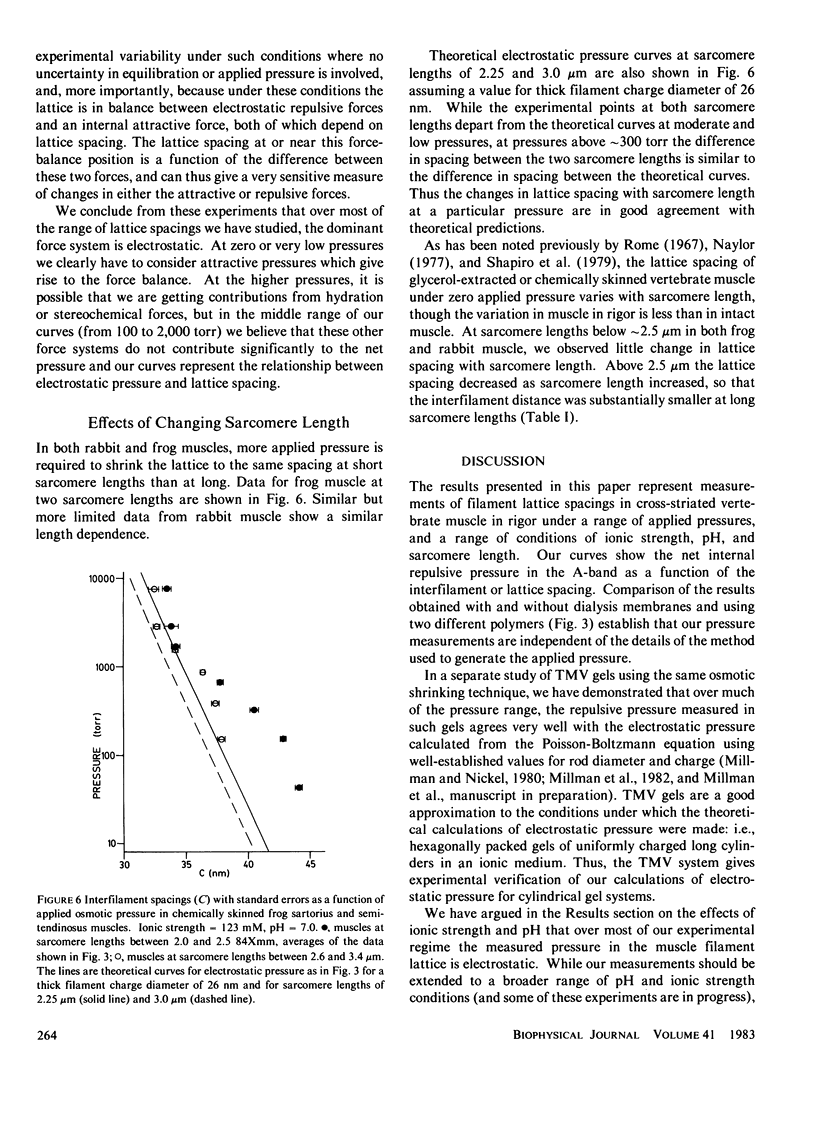
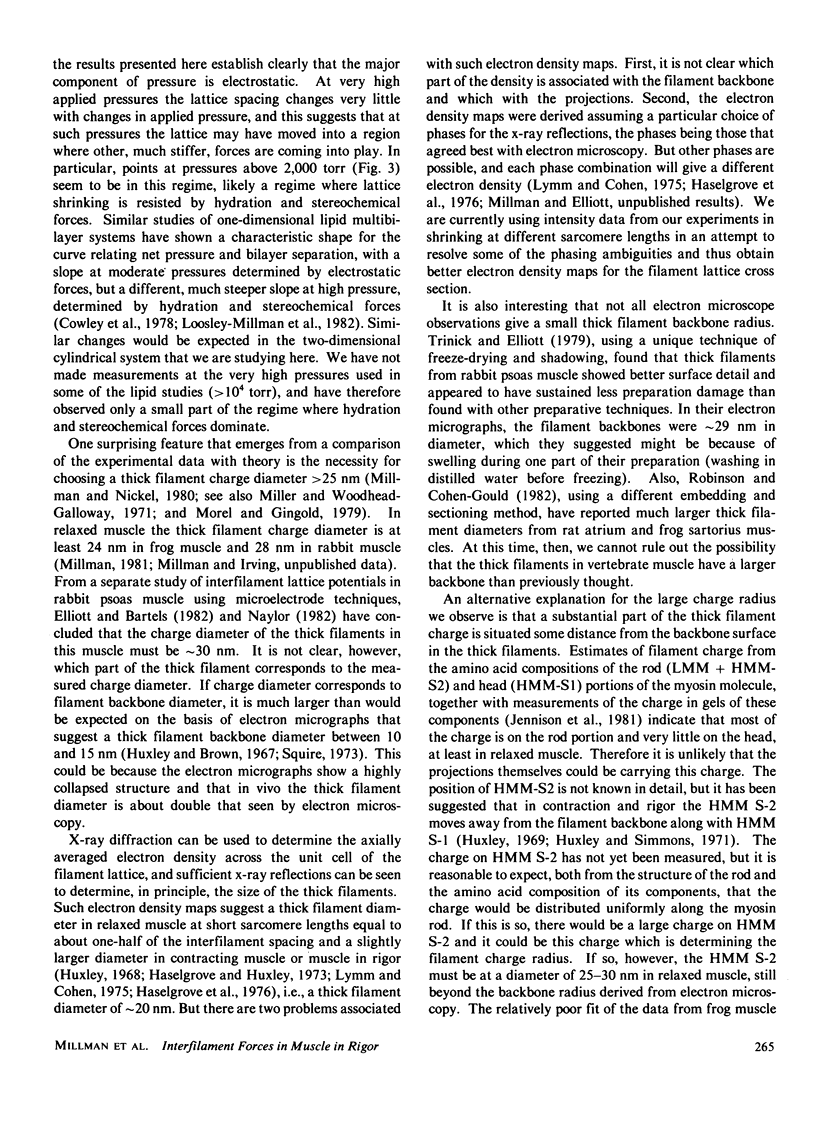
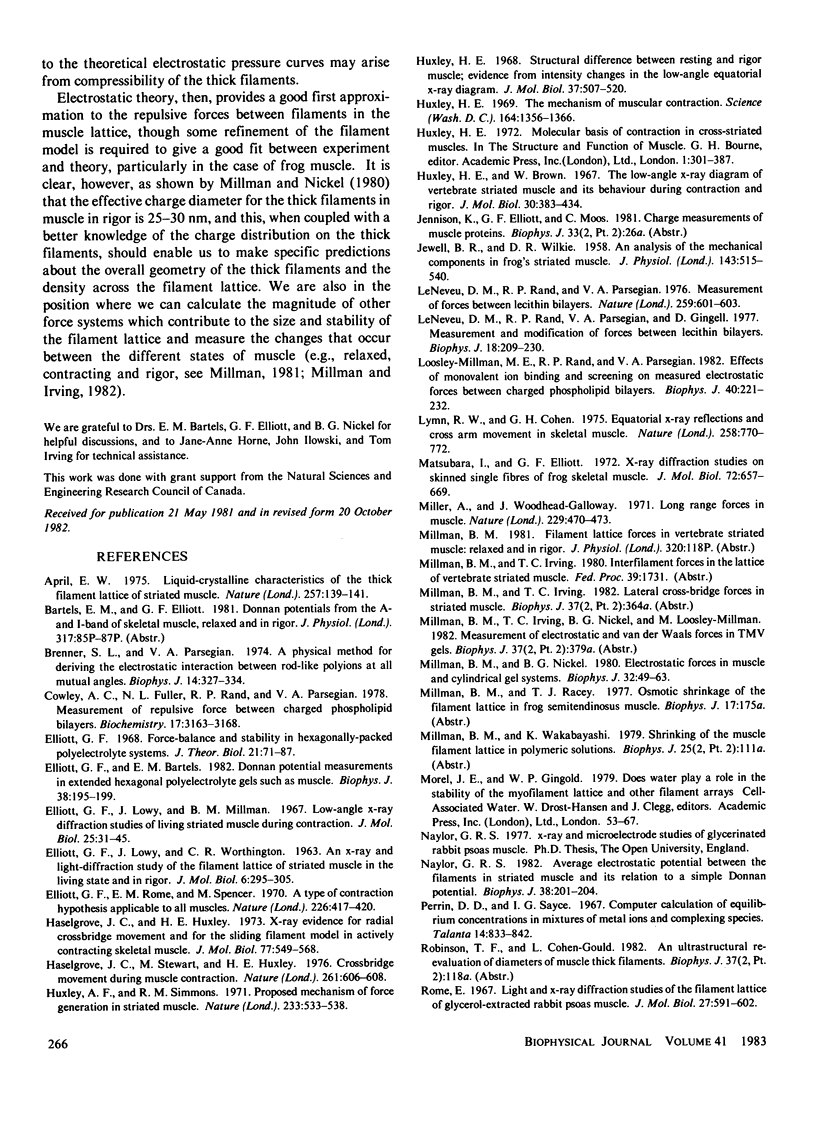
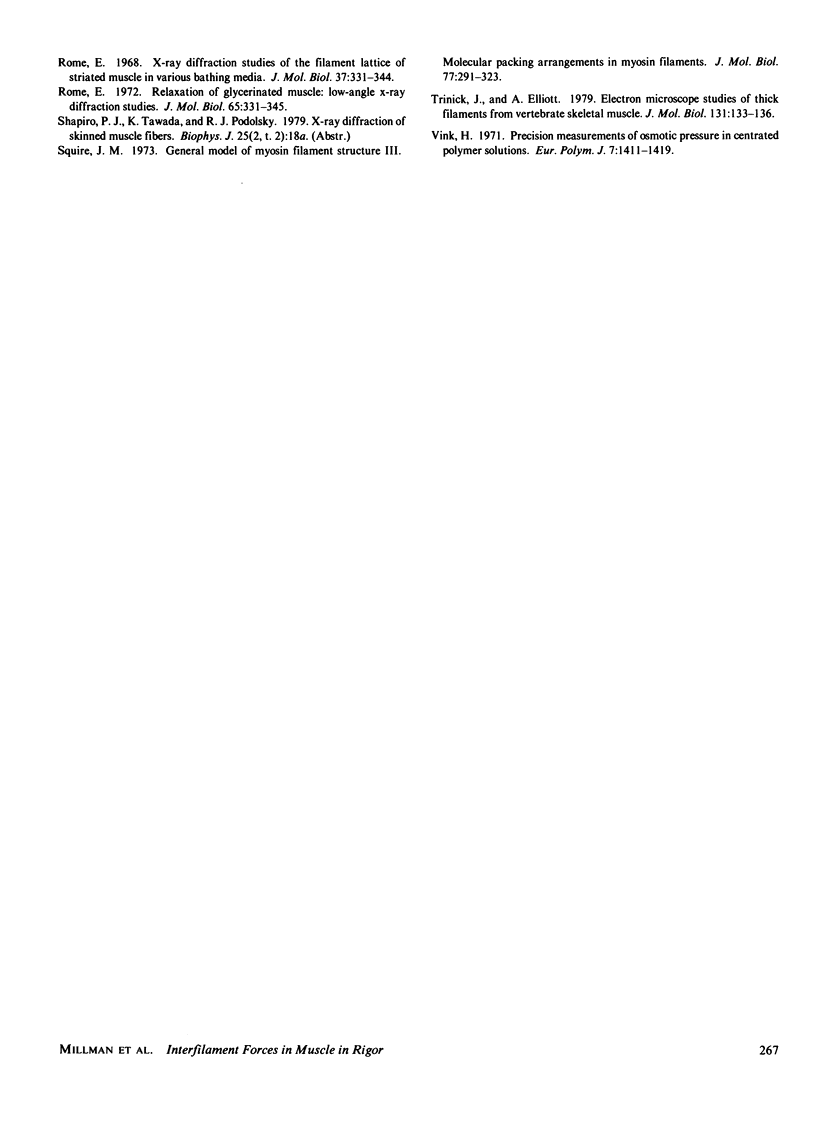
Selected References
These references are in PubMed. This may not be the complete list of references from this article.
- April E. W. Liquid-crystalline characteristics of the thick filament lattice of striated muscle. Nature. 1975 Sep 11;257(5522):139–141. doi: 10.1038/257139a0. [DOI] [PubMed] [Google Scholar]
- Brenner S. L., Parsegian V. A. A physical method for deriving the electrostatic interaction between rod-like polyions at all mutual angles. Biophys J. 1974 Apr;14(4):327–334. doi: 10.1016/S0006-3495(74)85919-9. [DOI] [PMC free article] [PubMed] [Google Scholar]
- Cowley A. C., Fuller N. L., Rand R. P., Parsegian V. A. Measurement of repulsive forces between charged phospholipid bilayers. Biochemistry. 1978 Jul 25;17(15):3163–3168. doi: 10.1021/bi00608a034. [DOI] [PubMed] [Google Scholar]
- Elliott G. F., Bartels E. M. Donnan potential measurements in extended hexagonal polyelectrolyte gels such as muscle. Biophys J. 1982 May;38(2):195–199. doi: 10.1016/S0006-3495(82)84546-3. [DOI] [PMC free article] [PubMed] [Google Scholar]
- Elliott G. F. Force-balances and stability in hexagonally-packed polyelectrolyte systems. J Theor Biol. 1968 Oct;21(1):71–87. doi: 10.1016/0022-5193(68)90060-x. [DOI] [PubMed] [Google Scholar]
- Elliott G. F., Lowy J., Millman B. M. Low-angle x-ray diffraction studies of living striated muscle during contraction. J Mol Biol. 1967 Apr 14;25(1):31–45. doi: 10.1016/0022-2836(67)90277-x. [DOI] [PubMed] [Google Scholar]
- Elliott G. F., Rome E. M., Spencer M. A type of contraction hypothesis applicable to all muscles. Nature. 1970 May 2;226(5244):417–420. doi: 10.1038/226417a0. [DOI] [PubMed] [Google Scholar]
- Haselgrove J. C., Huxley H. E. X-ray evidence for radial cross-bridge movement and for the sliding filament model in actively contracting skeletal muscle. J Mol Biol. 1973 Jul 15;77(4):549–568. doi: 10.1016/0022-2836(73)90222-2. [DOI] [PubMed] [Google Scholar]
- Haselgrove J. C., Stewart M., Huxley H. E. Cross-bridge movement during muscle contraction. Nature. 1976 Jun 17;261(5561):606–608. doi: 10.1038/261606a0. [DOI] [PubMed] [Google Scholar]
- Huxley A. F., Simmons R. M. Proposed mechanism of force generation in striated muscle. Nature. 1971 Oct 22;233(5321):533–538. doi: 10.1038/233533a0. [DOI] [PubMed] [Google Scholar]
- Huxley H. E., Brown W. The low-angle x-ray diagram of vertebrate striated muscle and its behaviour during contraction and rigor. J Mol Biol. 1967 Dec 14;30(2):383–434. doi: 10.1016/s0022-2836(67)80046-9. [DOI] [PubMed] [Google Scholar]
- Huxley H. E. Structural difference between resting and rigor muscle; evidence from intensity changes in the lowangle equatorial x-ray diagram. J Mol Biol. 1968 Nov 14;37(3):507–520. doi: 10.1016/0022-2836(68)90118-6. [DOI] [PubMed] [Google Scholar]
- Huxley H. E. The mechanism of muscular contraction. Science. 1969 Jun 20;164(3886):1356–1365. doi: 10.1126/science.164.3886.1356. [DOI] [PubMed] [Google Scholar]
- JEWELL B. R., WILKIE D. R. An analysis of the mechanical components in frog's striated muscle. J Physiol. 1958 Oct 31;143(3):515–540. doi: 10.1113/jphysiol.1958.sp006075. [DOI] [PMC free article] [PubMed] [Google Scholar]
- LeNeveu D. M., Rand R. P. Measurement and modification of forces between lecithin bilayers. Biophys J. 1977 May;18(2):209–230. doi: 10.1016/S0006-3495(77)85608-7. [DOI] [PMC free article] [PubMed] [Google Scholar]
- LeNeveu D. M., Rand R. P., Parsegian V. A. Measurement of forces between lecithin bilayers. Nature. 1976 Feb 19;259(5544):601–603. doi: 10.1038/259601a0. [DOI] [PubMed] [Google Scholar]
- Loosley-Millman M. E., Rand R. P., Parsegian V. A. Effects of monovalent ion binding and screening on measured electrostatic forces between charged phospholipid bilayers. Biophys J. 1982 Dec;40(3):221–232. doi: 10.1016/S0006-3495(82)84477-9. [DOI] [PMC free article] [PubMed] [Google Scholar]
- Lymn R. W. Equatorial X-ray reflections and cross arm movement in skeletal muscle. Nature. 1975 Dec 25;258(5537):770–772. doi: 10.1038/258770a0. [DOI] [PubMed] [Google Scholar]
- Matsubara I., Elliott G. F. X-ray diffraction studies on skinned single fibres of frog skeletal muscle. J Mol Biol. 1972 Dec 30;72(3):657–669. doi: 10.1016/0022-2836(72)90183-0. [DOI] [PubMed] [Google Scholar]
- Miller A., Woodhead-Galloway J. Long range forces in muscle. Nature. 1971 Feb 12;229(5285):470–473. doi: 10.1038/229470a0. [DOI] [PubMed] [Google Scholar]
- Millman B. M., Nickel B. G. Electrostatic forces in muscle and cylindrical gel systems. Biophys J. 1980 Oct;32(1):49–63. doi: 10.1016/S0006-3495(80)84915-0. [DOI] [PMC free article] [PubMed] [Google Scholar]
- Naylor G. R. Average electrostatic potential between the filaments in striated muscle and its relation to a simple Donnan potential. Biophys J. 1982 May;38(2):201–204. doi: 10.1016/S0006-3495(82)84547-5. [DOI] [PMC free article] [PubMed] [Google Scholar]
- Rome E. Light and X-ray diffraction studies of the filament lattice of glycerol-extracted rabbit psoas muscle. J Mol Biol. 1967 Aug 14;27(3):591–602. doi: 10.1016/0022-2836(67)90061-7. [DOI] [PubMed] [Google Scholar]
- Rome E. Relaxation of glycerinated muscle: low-angle x-ray diffraction studies. J Mol Biol. 1972 Mar 28;65(2):331–345. doi: 10.1016/0022-2836(72)90285-9. [DOI] [PubMed] [Google Scholar]
- Rome E. X-ray diffraction studies of the filament lattice of striated muscle in various bathing media. J Mol Biol. 1968 Oct 28;37(2):331–344. doi: 10.1016/0022-2836(68)90272-6. [DOI] [PubMed] [Google Scholar]
- Squire J. M. General model of myosin filament structure. 3. Molecular packing arrangements in myosin filaments. J Mol Biol. 1973 Jun 25;77(2):291–323. doi: 10.1016/0022-2836(73)90337-9. [DOI] [PubMed] [Google Scholar]
- Trinick J., Elliott A. Electron microscope studies of thick filaments from vertebrate skeletal muscle. J Mol Biol. 1979 Jun 15;131(1):133–136. doi: 10.1016/0022-2836(79)90304-8. [DOI] [PubMed] [Google Scholar]