Abstract
1. There are problems associated with the notion that slow potentials alone are used to transmit information in the early stages of some visual systems. This idea and alternatives have been tested on the barnacle lateral ocellus, a simple eye with only three photoreceptors, each with its own axon about 1 cm long.
2. All of the receptors have very similar properties including spectral sensitivity, and are also electrically coupled together. Impulses cannot be recorded from any of the cell bodies, all of which have been impaled as shown by dye marking.
3. No impulses can be recorded externally from most of the ocellar nerve or intracellularly from the receptor axon terminals. Impulses driven by light, sometimes recorded in the final part of the nerve, are believed to originate in other axons.
4. During illumination of the eye, current enters the receptor soma and leaves via the rest of the axon. This is consistent with the idea that the axon acts as a purely passive cable. The passive behaviour was also demonstrated in a comparison of the relative attenuation down the axon, of hyperpolarizations and depolarizations.
5. Calculations based on the supposed electrical constants of the somas showed that the slow potential itself was unlikely to be the visual signal, since it would be enormously attenuated by passive spread down the long thin axons. To check this, the axon terminals in the supraoesophageal ganglion were penetrated and identified by electrical and dye-marking criteria. In fact, the slow potential was attenuated in the most favourable case only by a factor of about three, indicating an axon membrane resistance in the range of 105 Ω. cm2.
6. This resistance may be substantially higher than that of the soma surface membrane, corrected for increased surface area. The sheath around each axon probably does not influence the electrical properties, judged by its permeability to the small molecule of Procion Yellow.
7. The minimal loss of voltage in the axon and the absence of regenerative activity implicate the slow potential itself as the visual signal. But there remains the alternative that light triggers some unknown transmission process, of which the slow potential is only an incidental by-product. If this were so, artificially imposed changes of membrane potential should not duplicate the action of light in promoting synaptic transmission. To test this, receptors were polarized by currents through the pipette whilst visually driven post-synaptic cells in the oesophageal connectives were being monitored. Currents could effectively substitute for lights to produce post-synaptic impulse trains of similar form and latency, confirming that the potential change produced by light is the normal visual signal.
8. Only increases of receptor membrane potential stimulate the particular post-synaptic axons examined, which give `off' responses to light. Transmission from the receptors is a voltage-dependent process which is most sensitive when a receptor is hyperpolarized from an already depolarized level.
9. The discrimination of small visual signals from intrinsic axon noise is discussed, and should pose no problem in the case of the barnacle, where the smallest effective signal measured was about 0·3 mV in the soma. In other eyes where the problem may be more severe, electrical junctions between receptors could significantly improve the signal/noise ratio.
Full text
PDF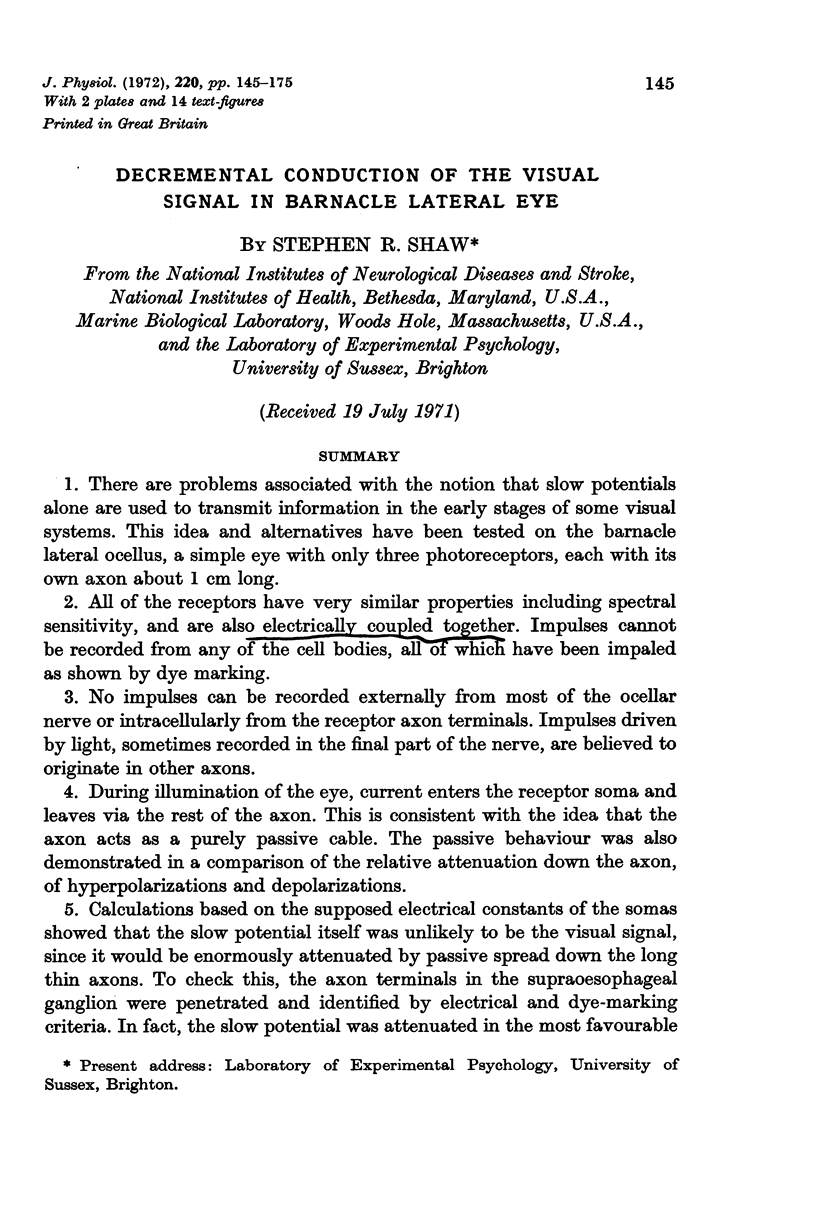
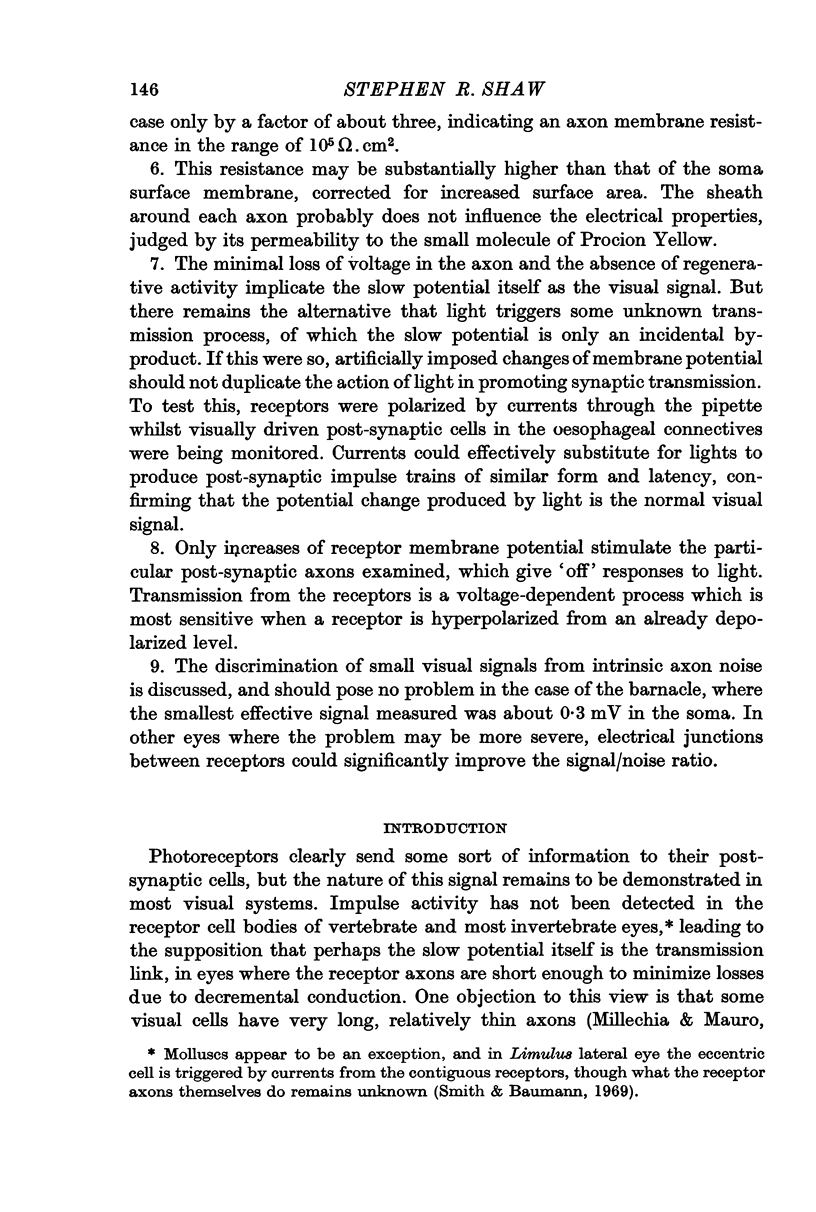
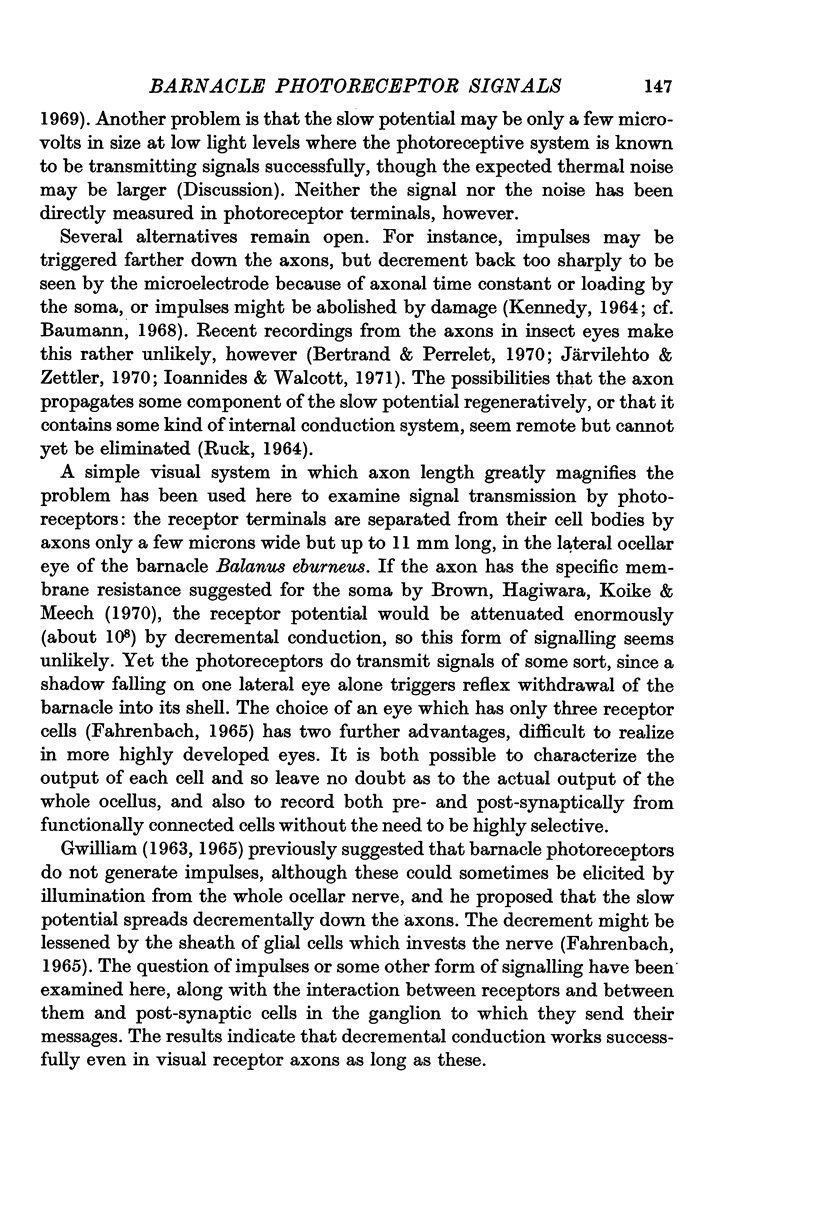
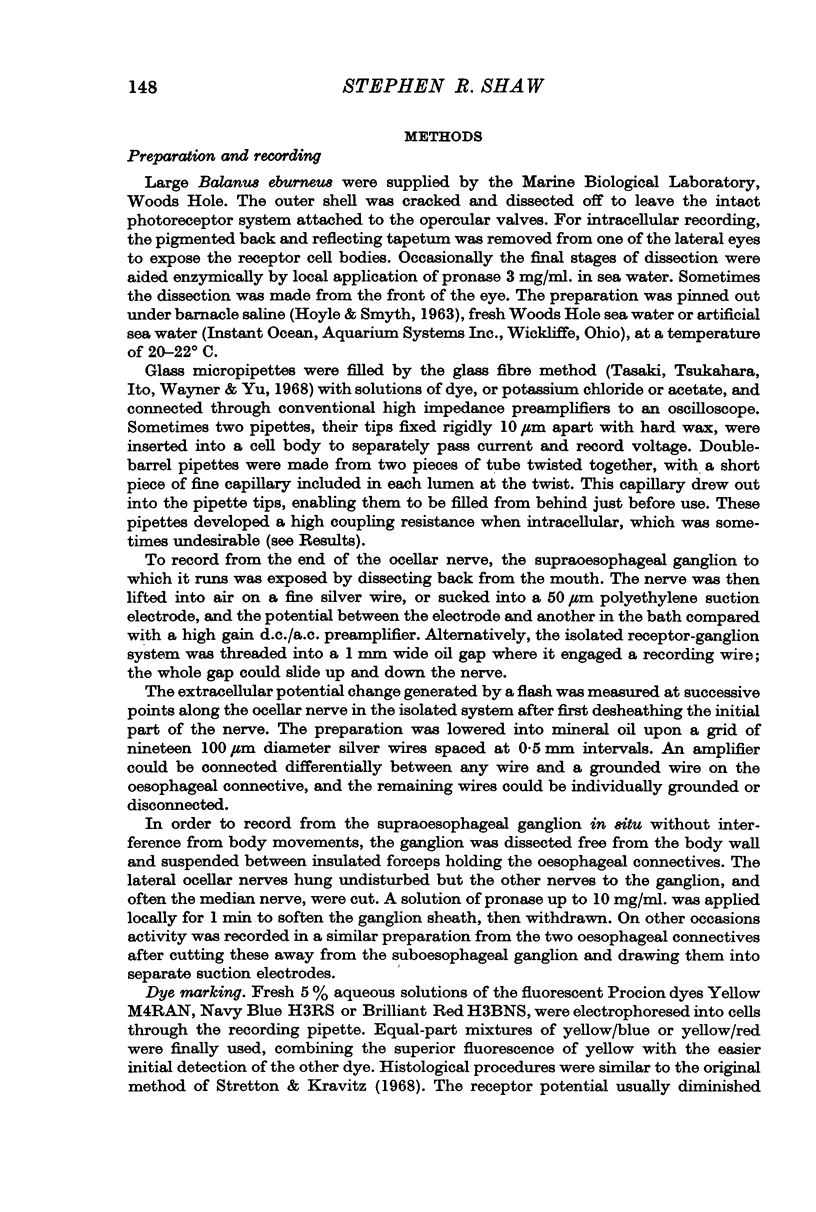
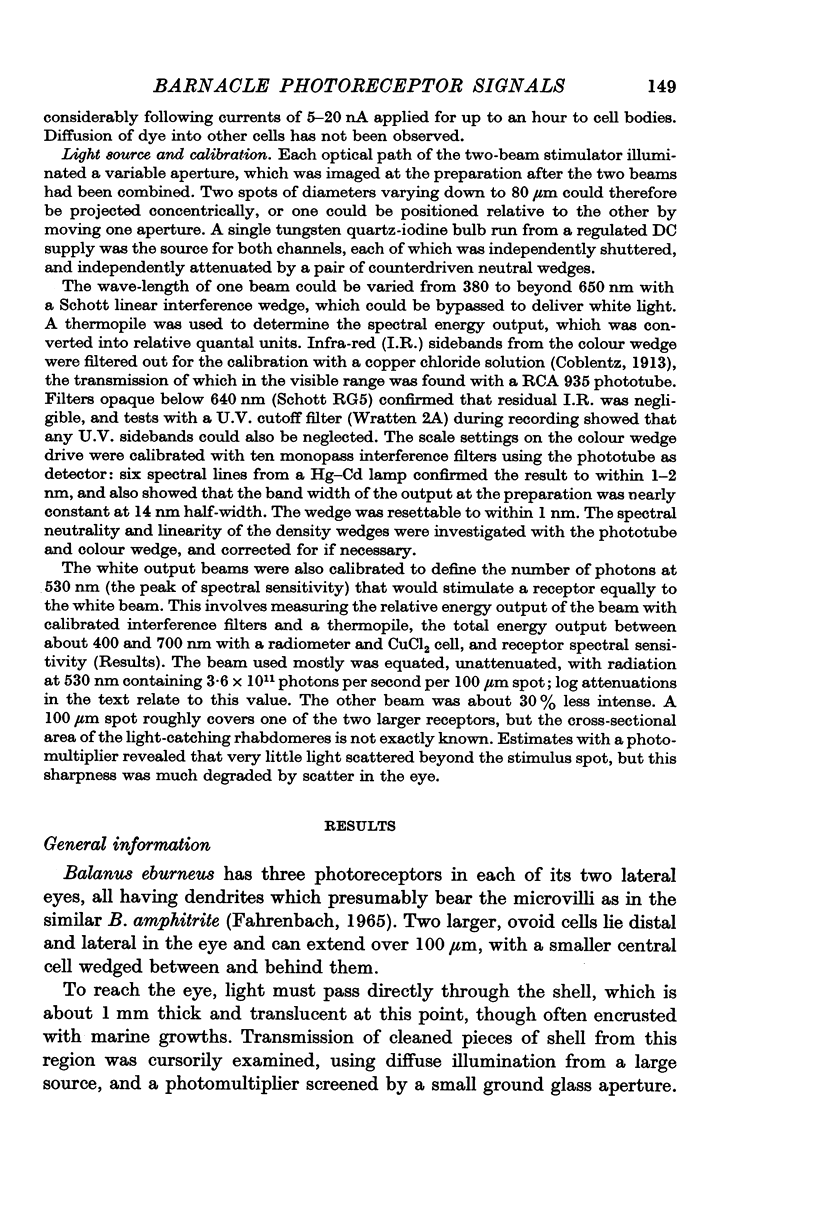
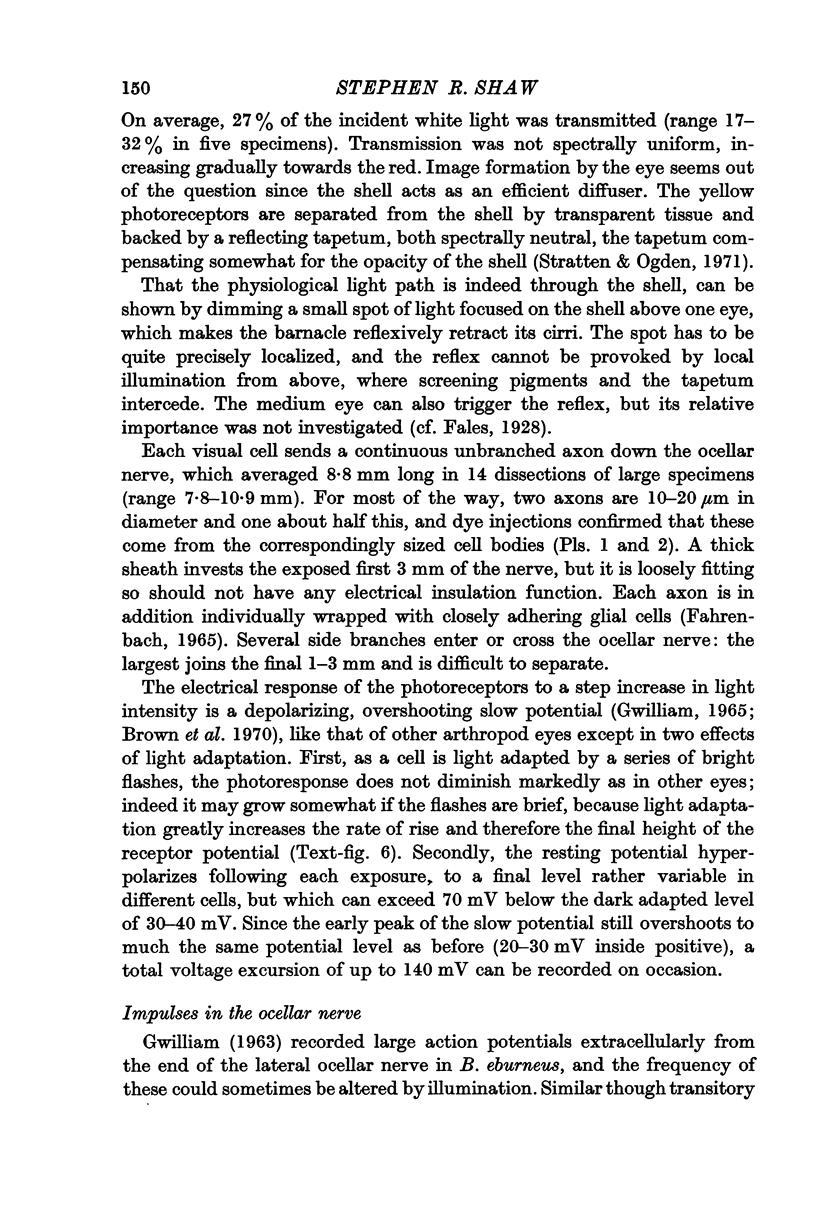
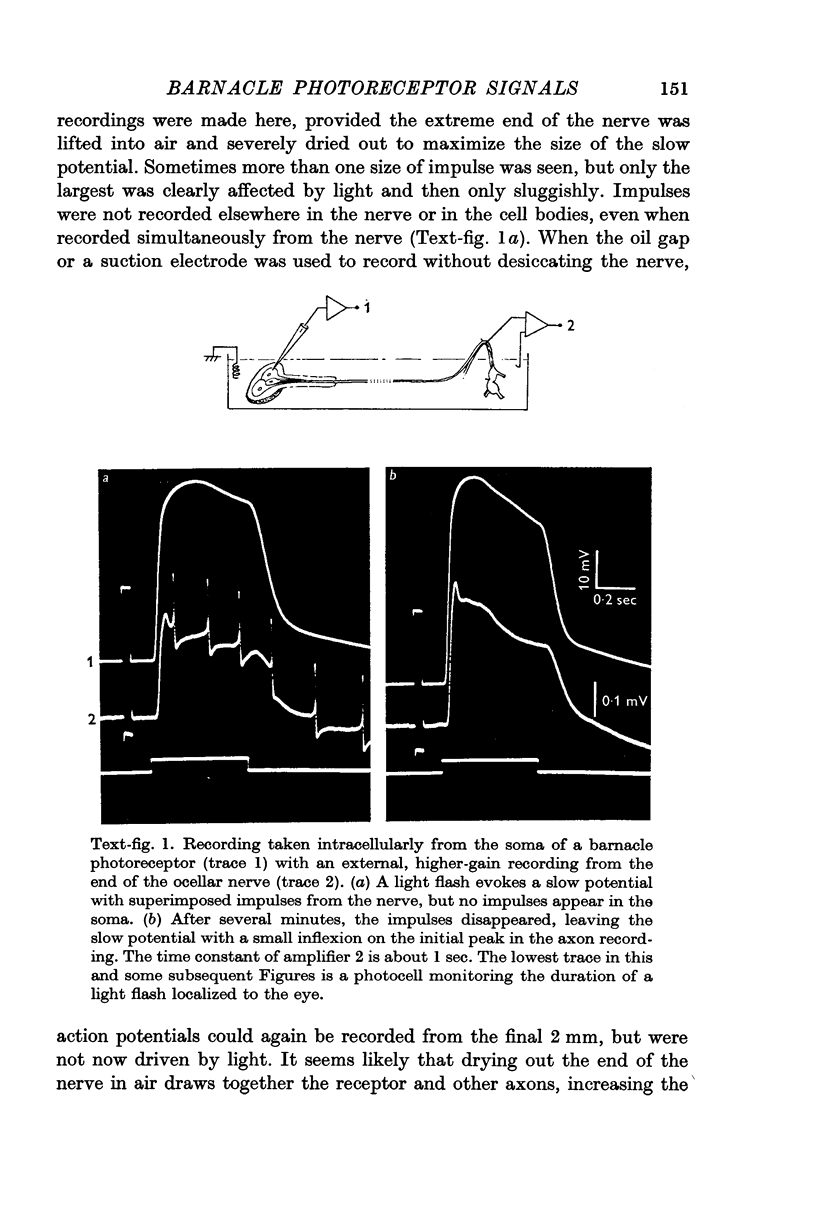
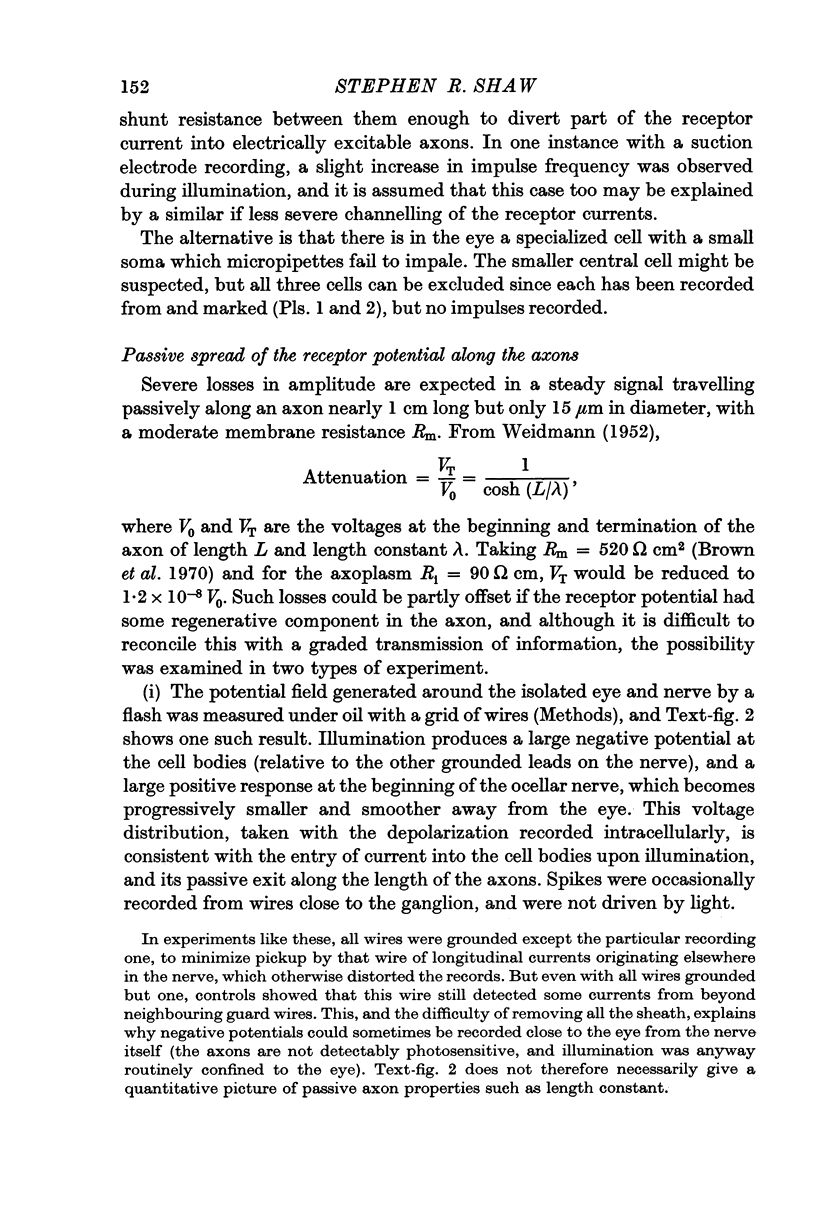
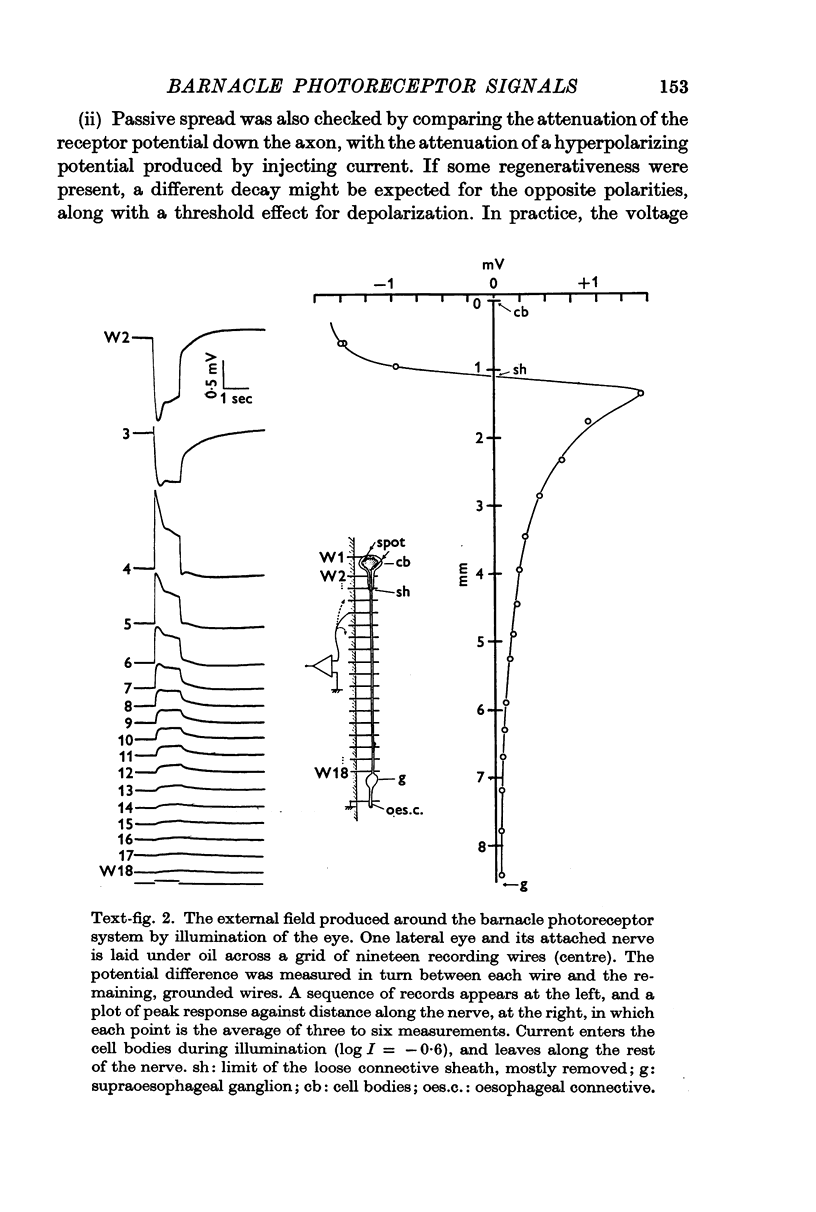
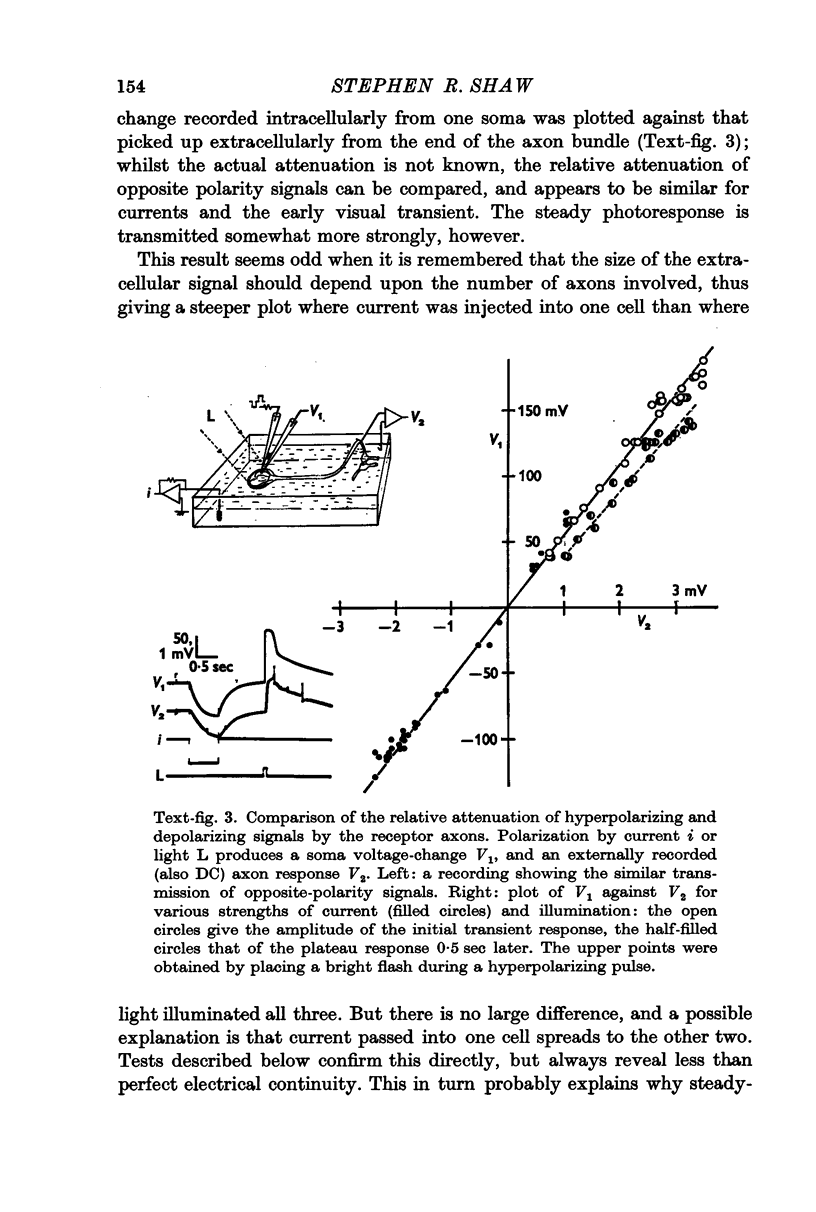
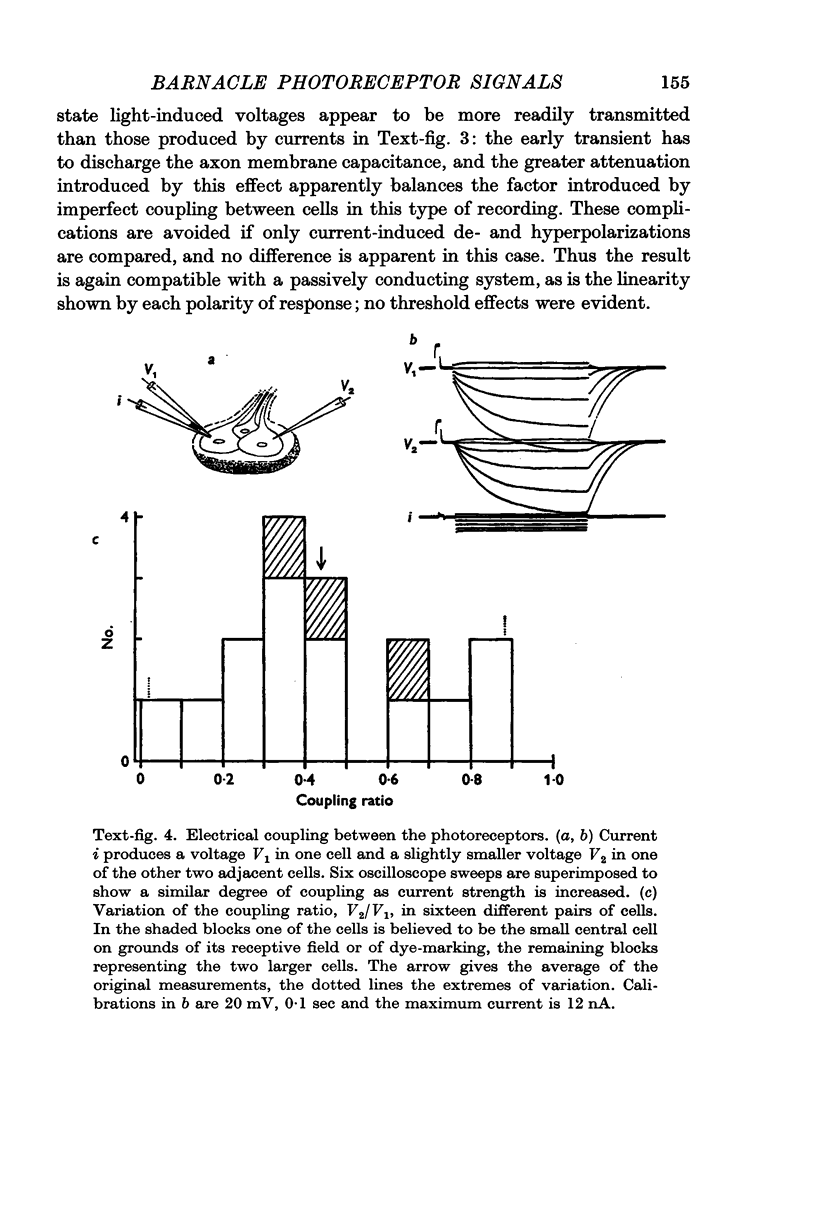
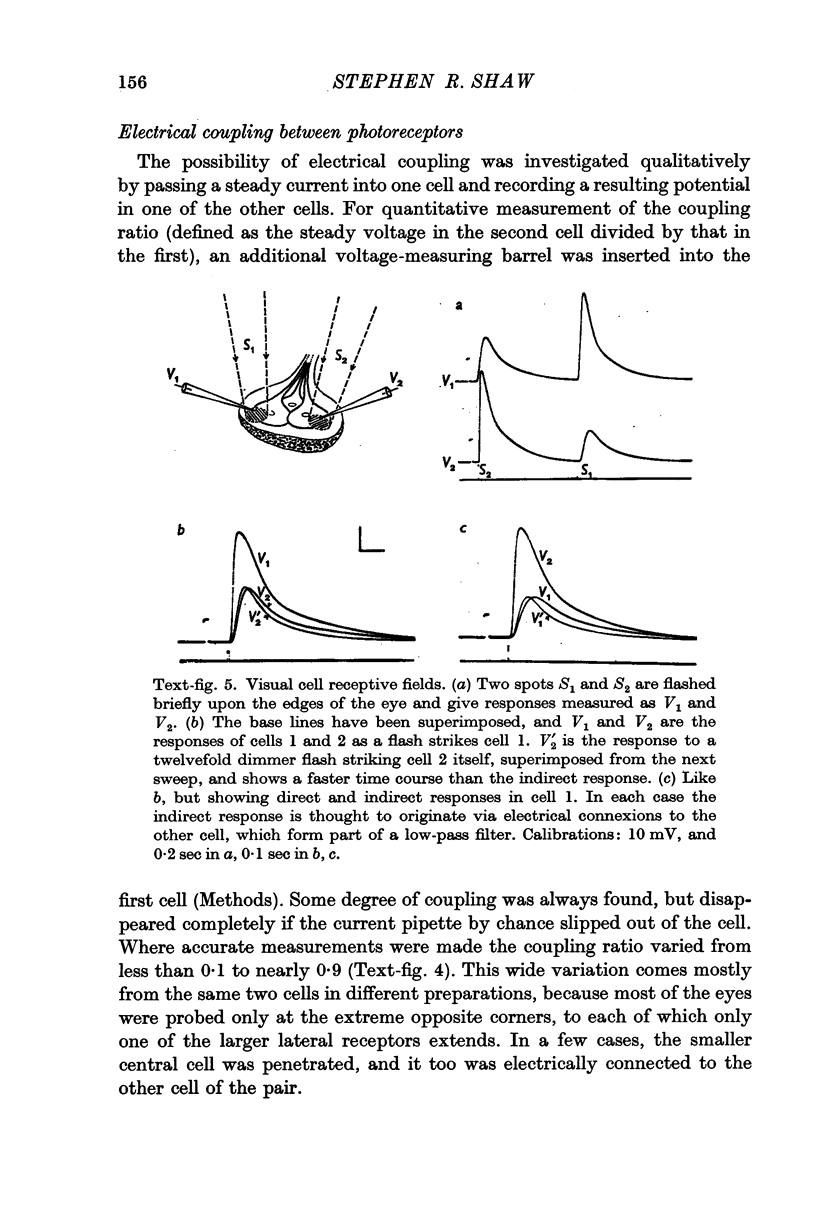
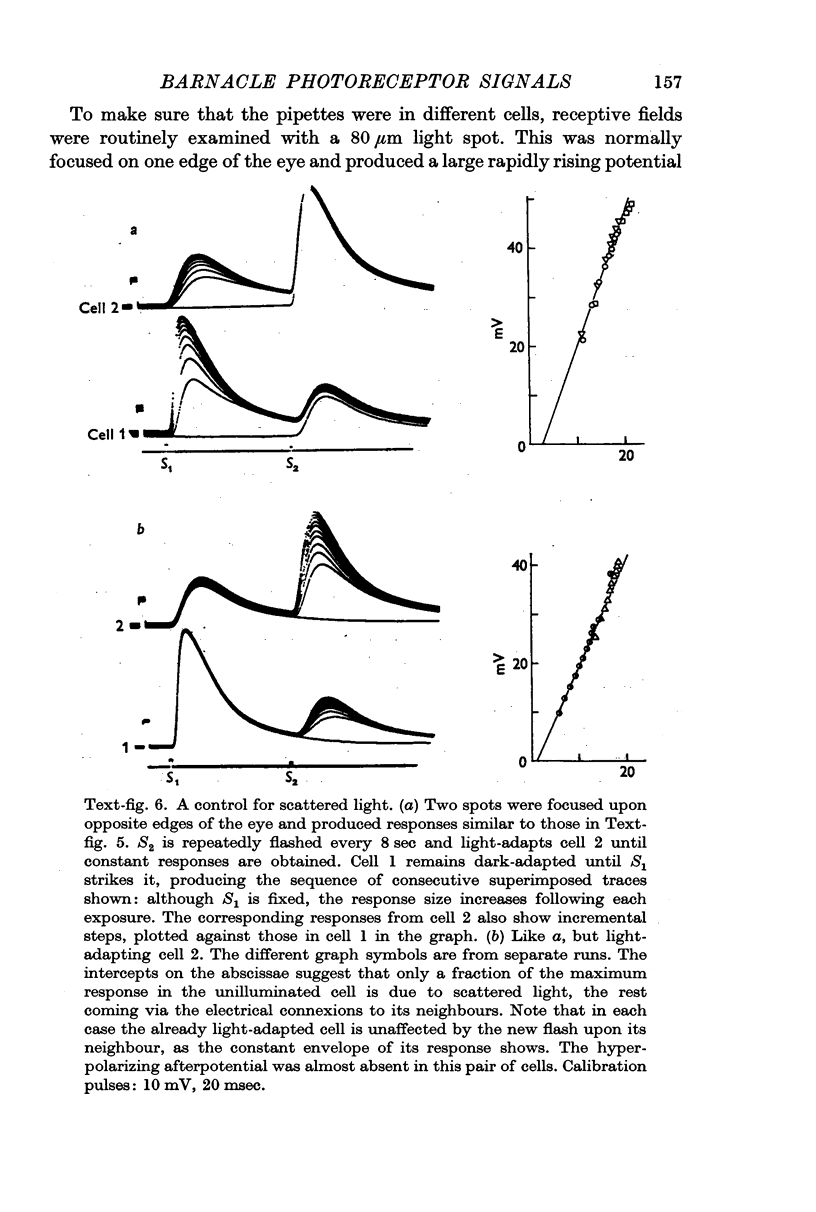
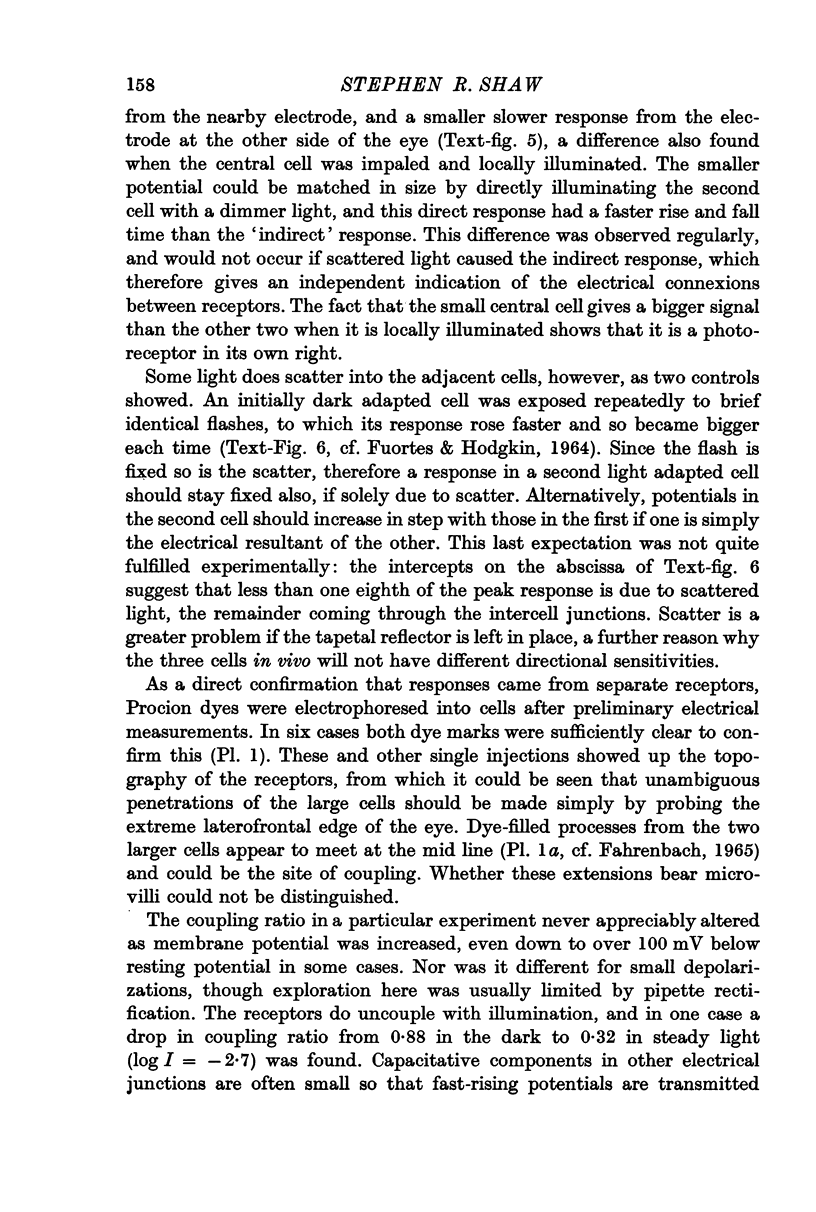
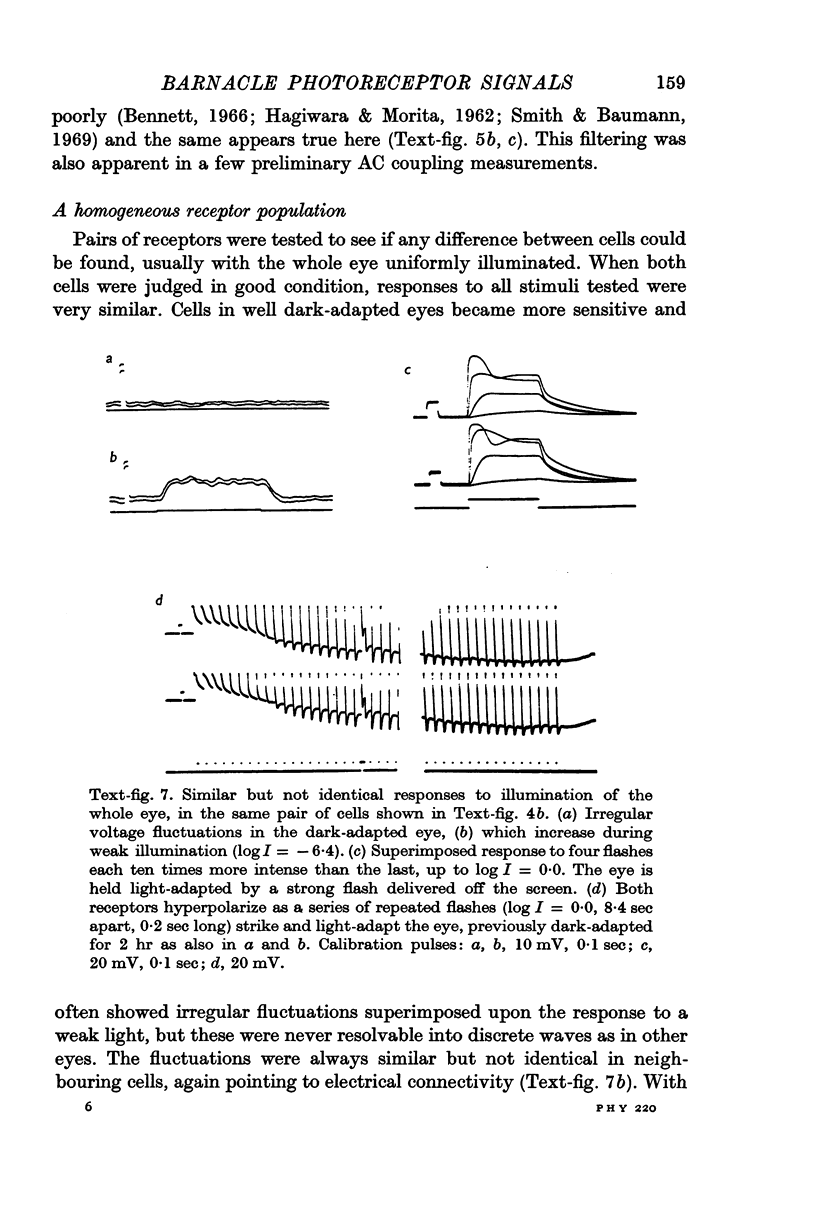
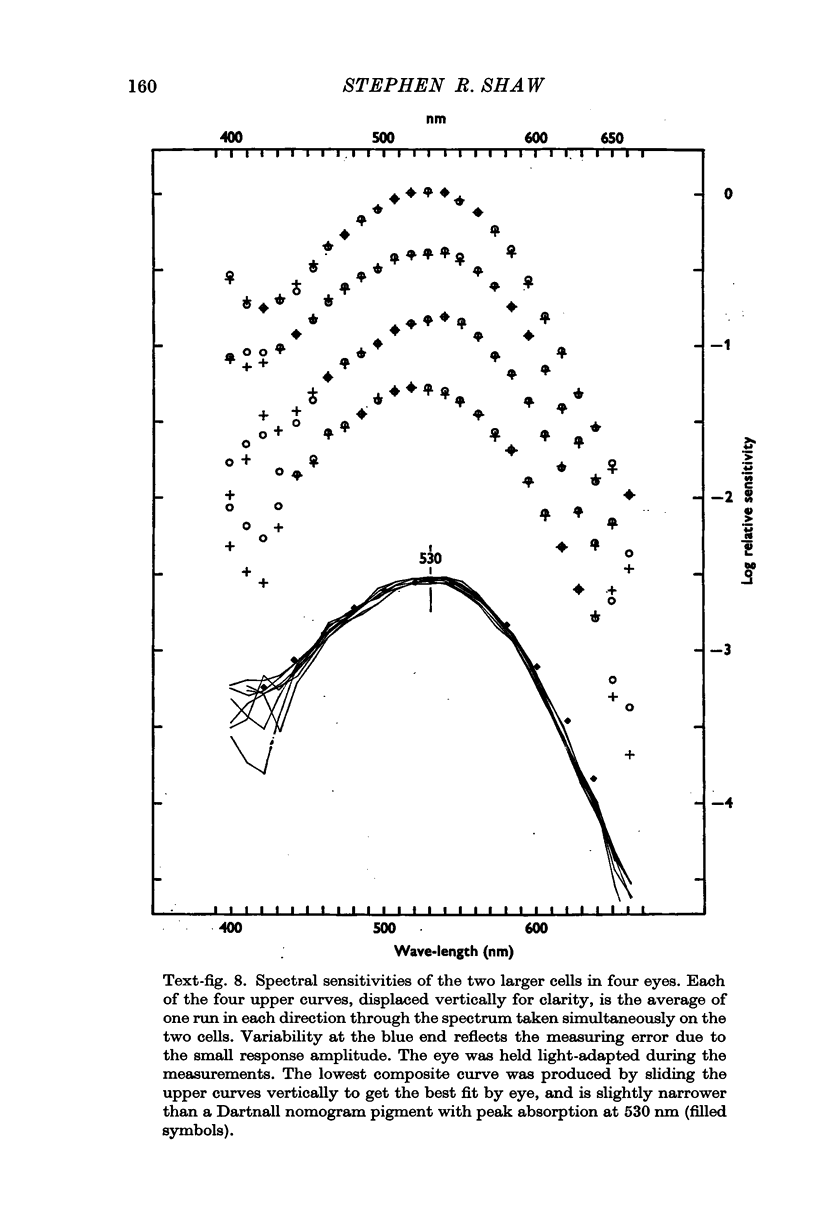
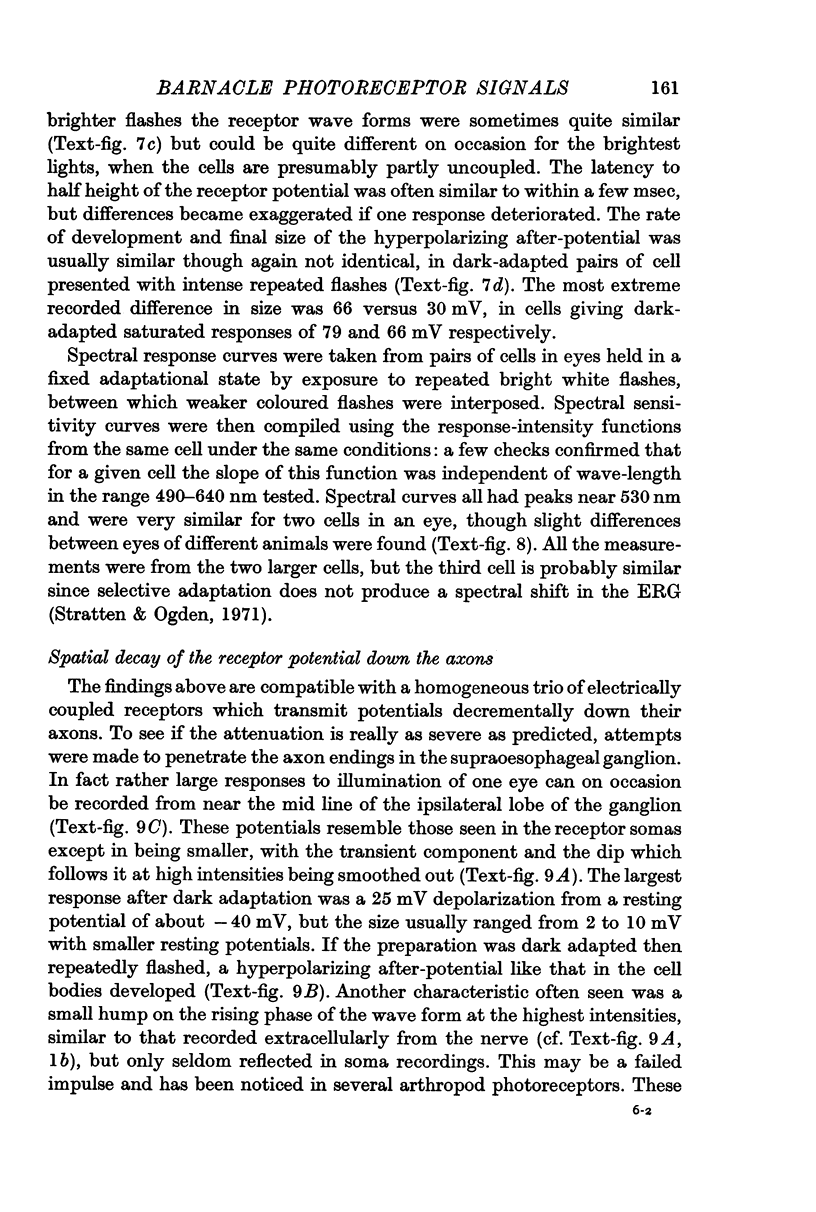
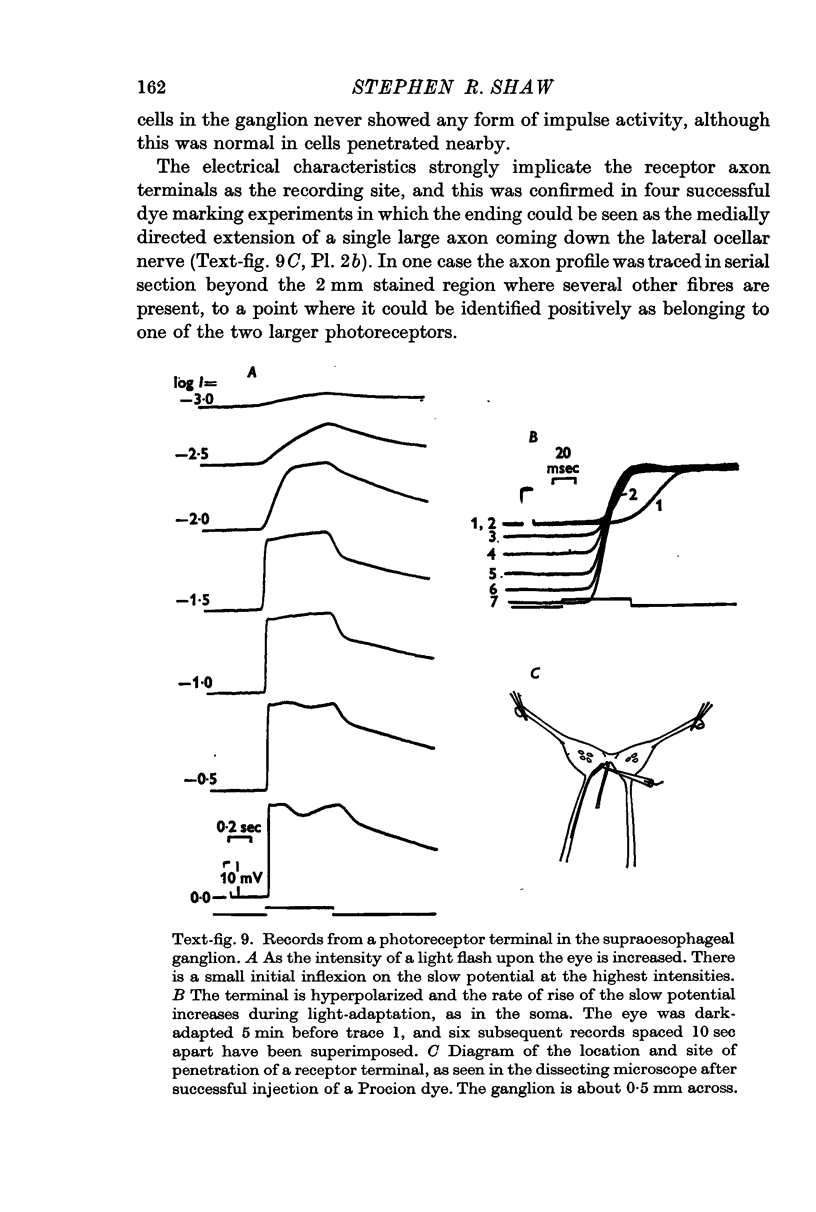
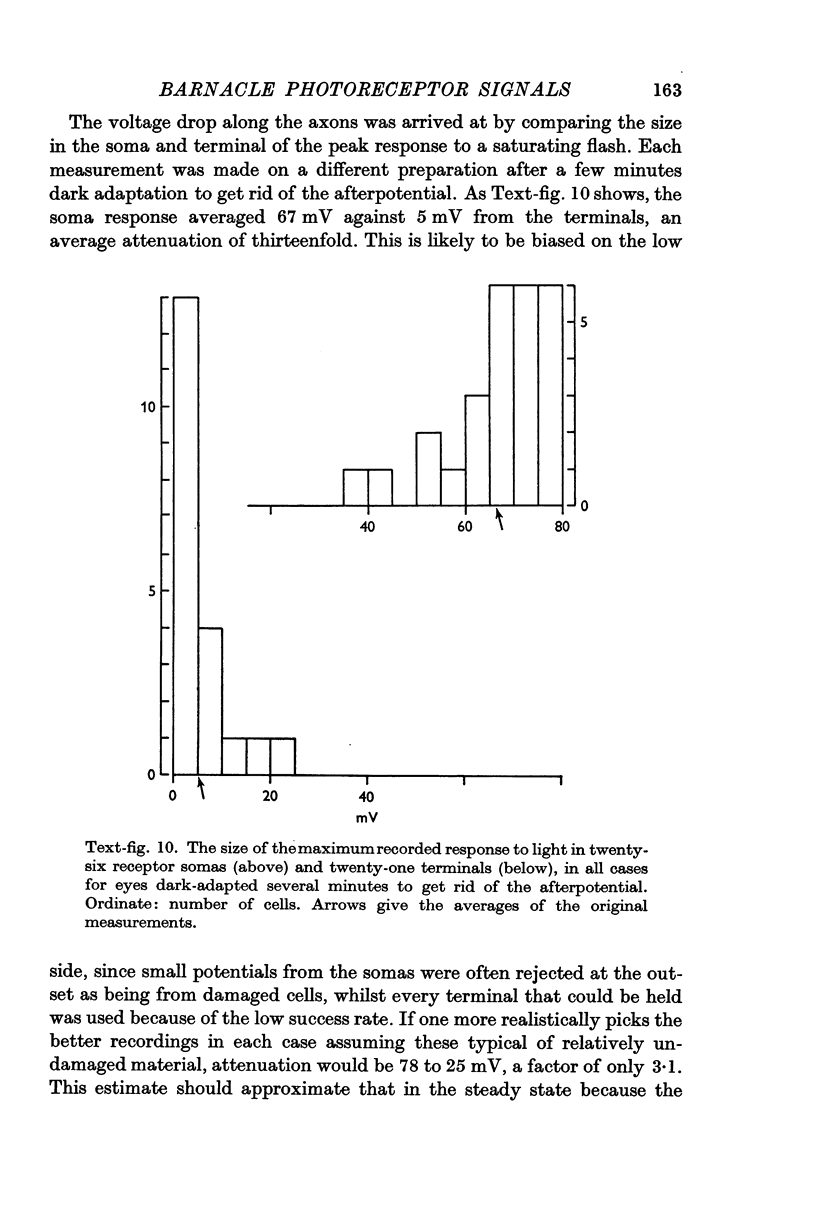
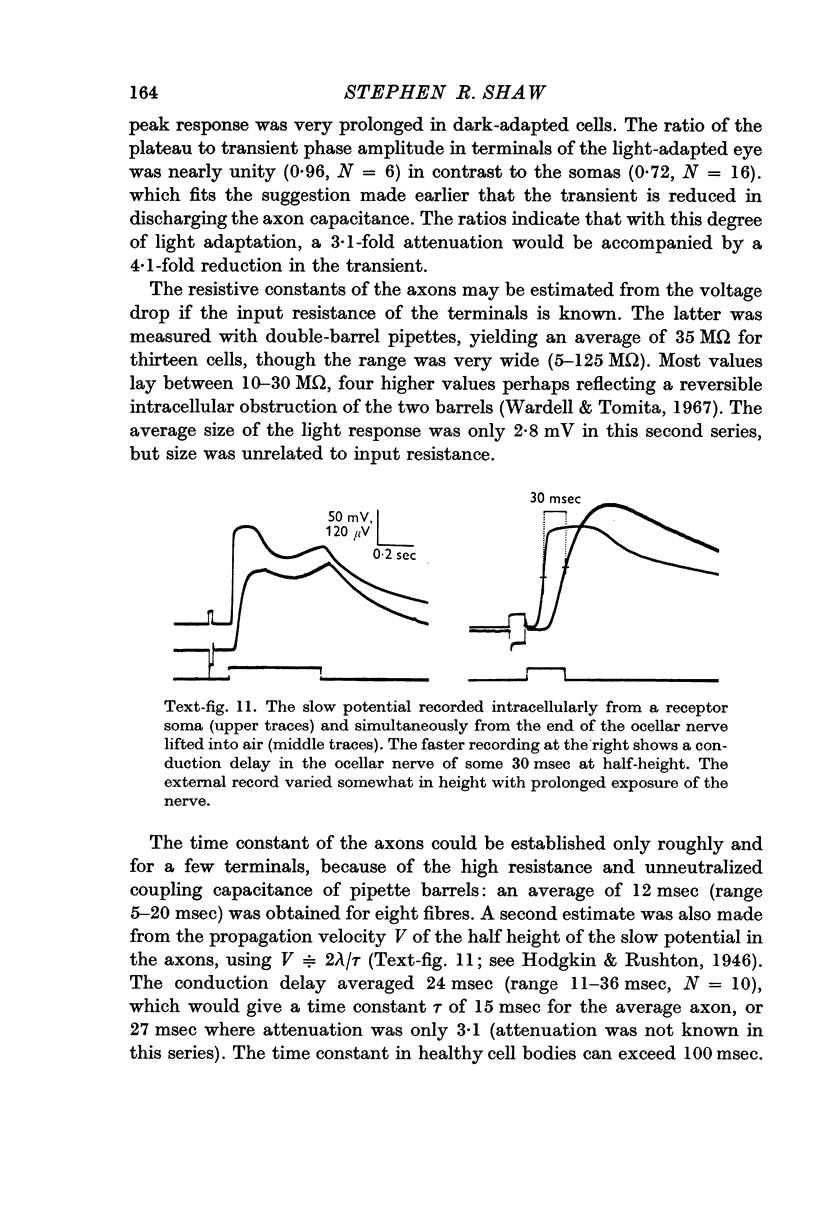
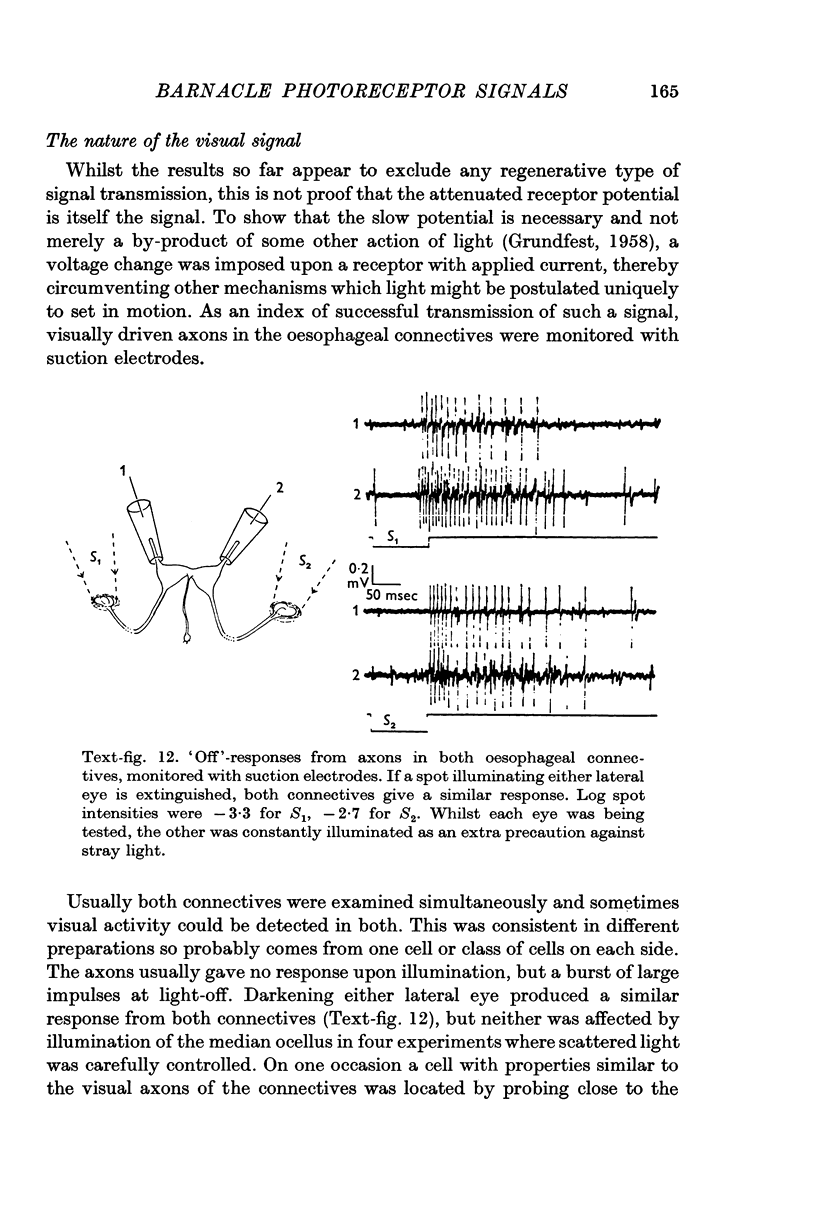
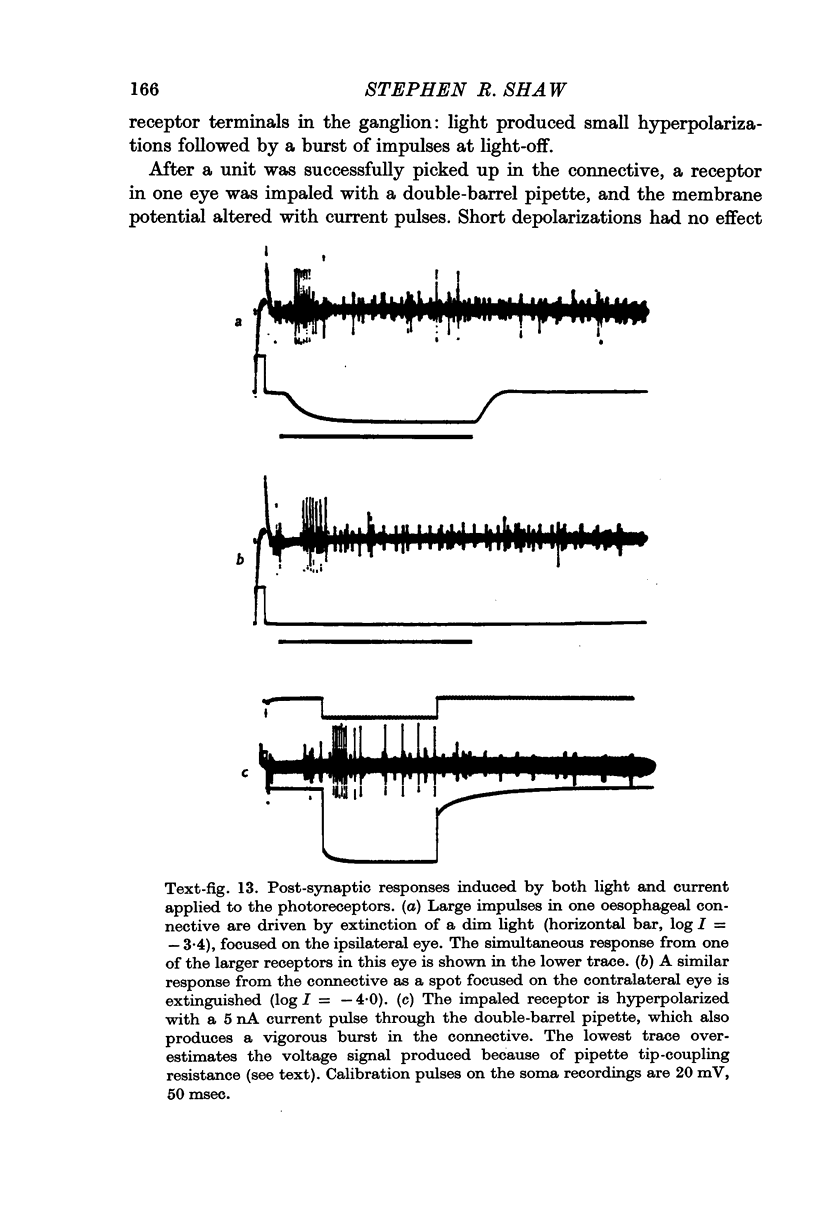
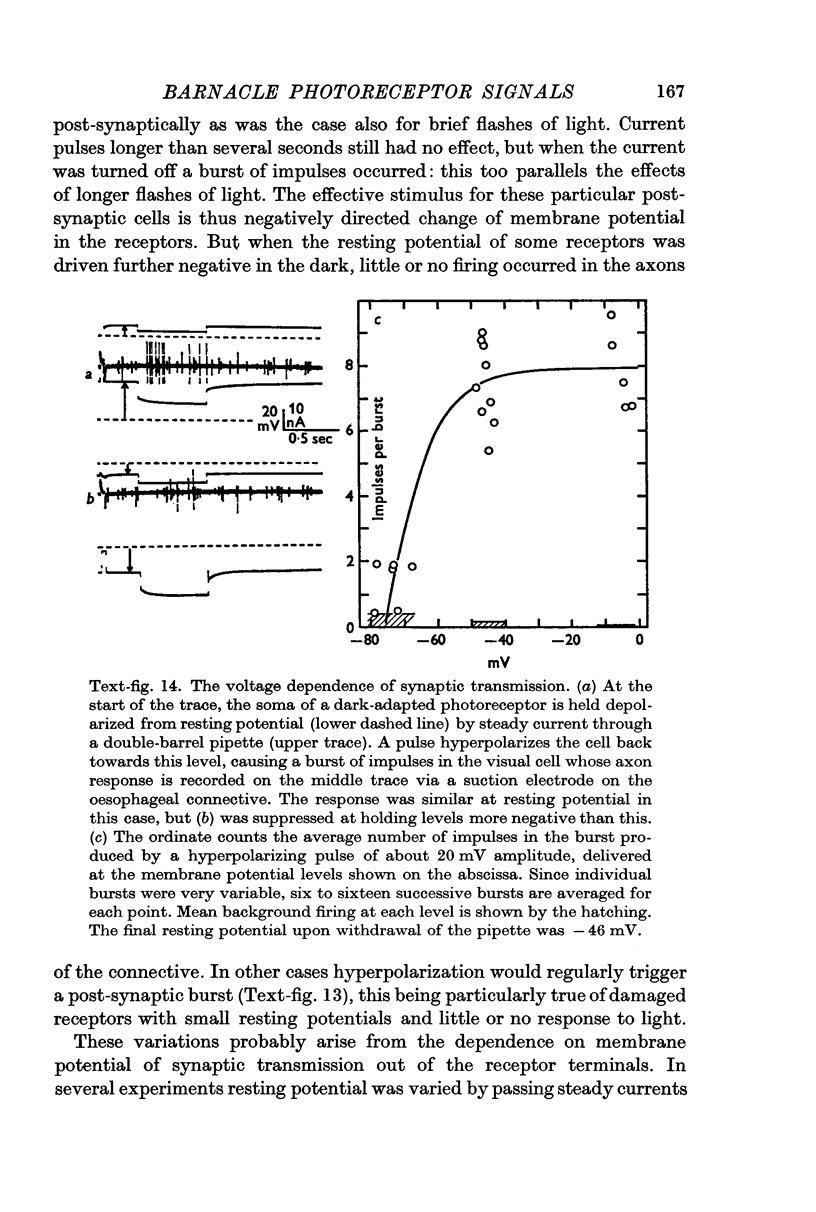
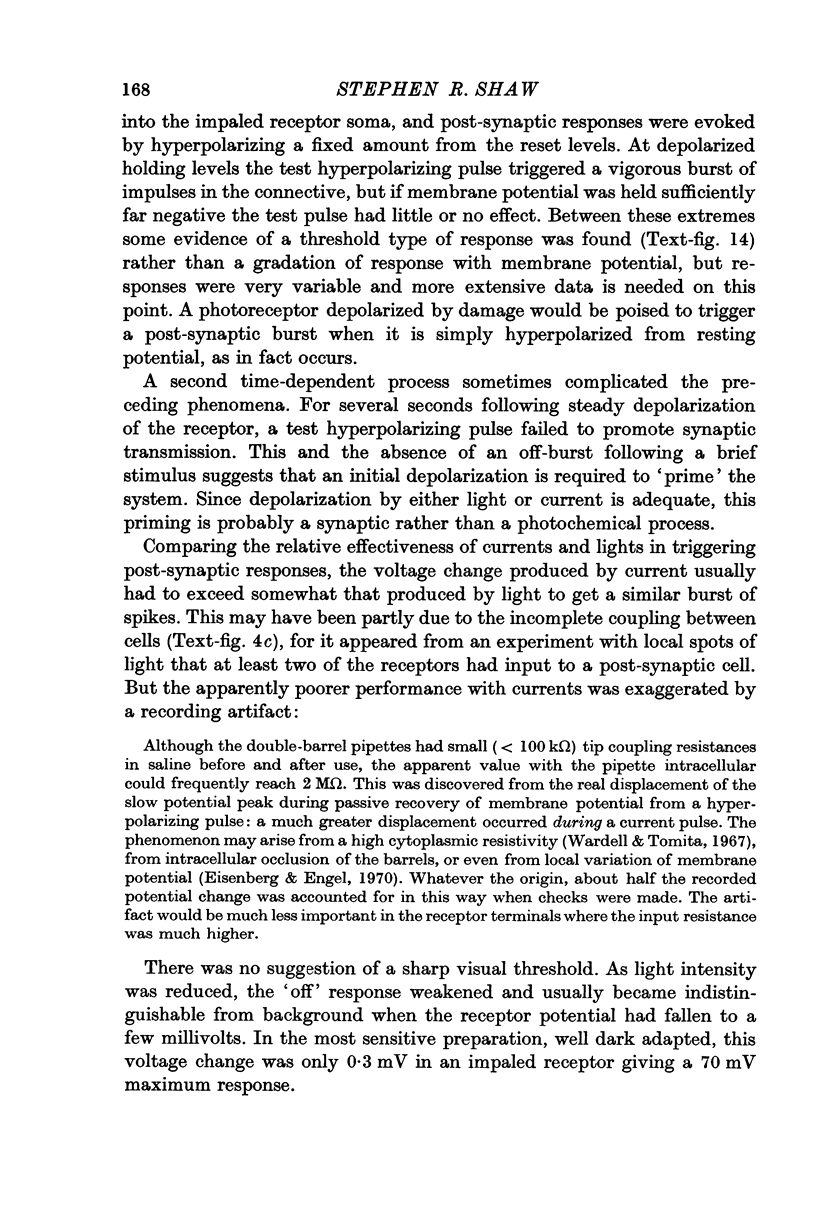
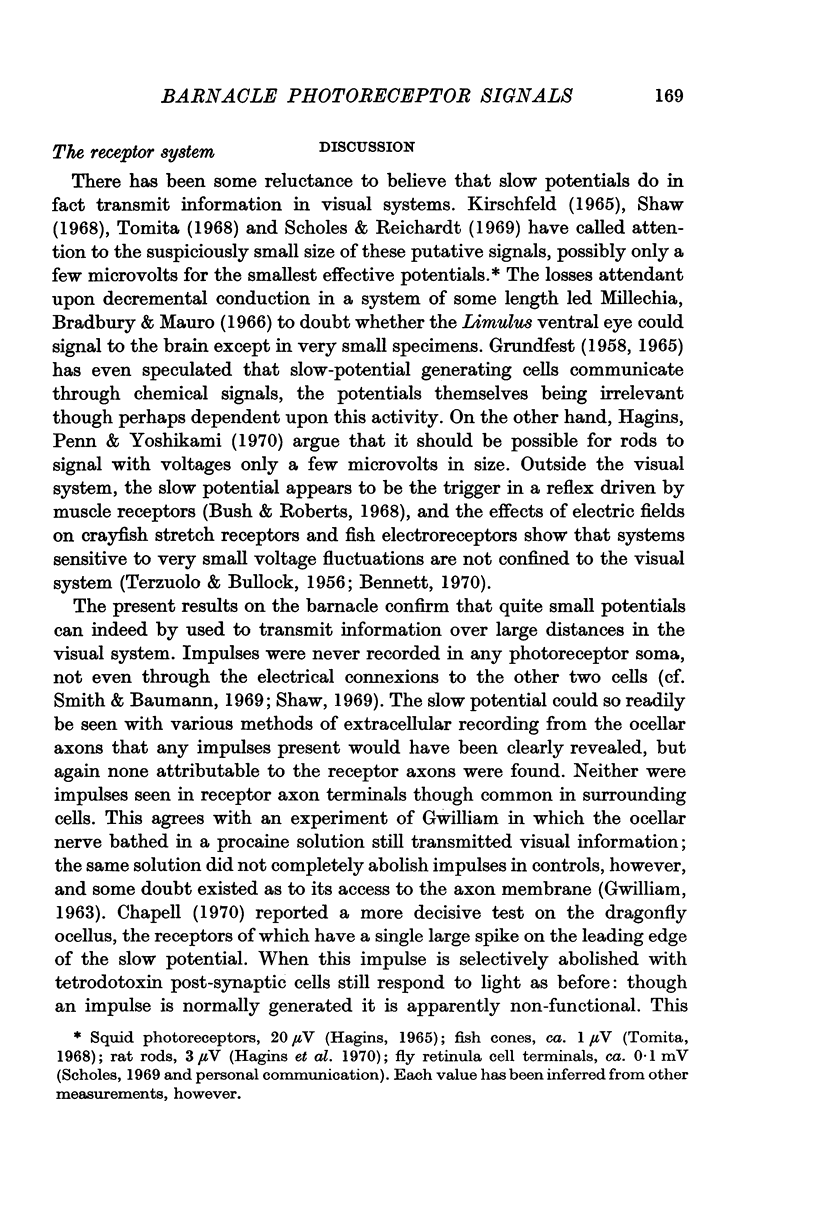
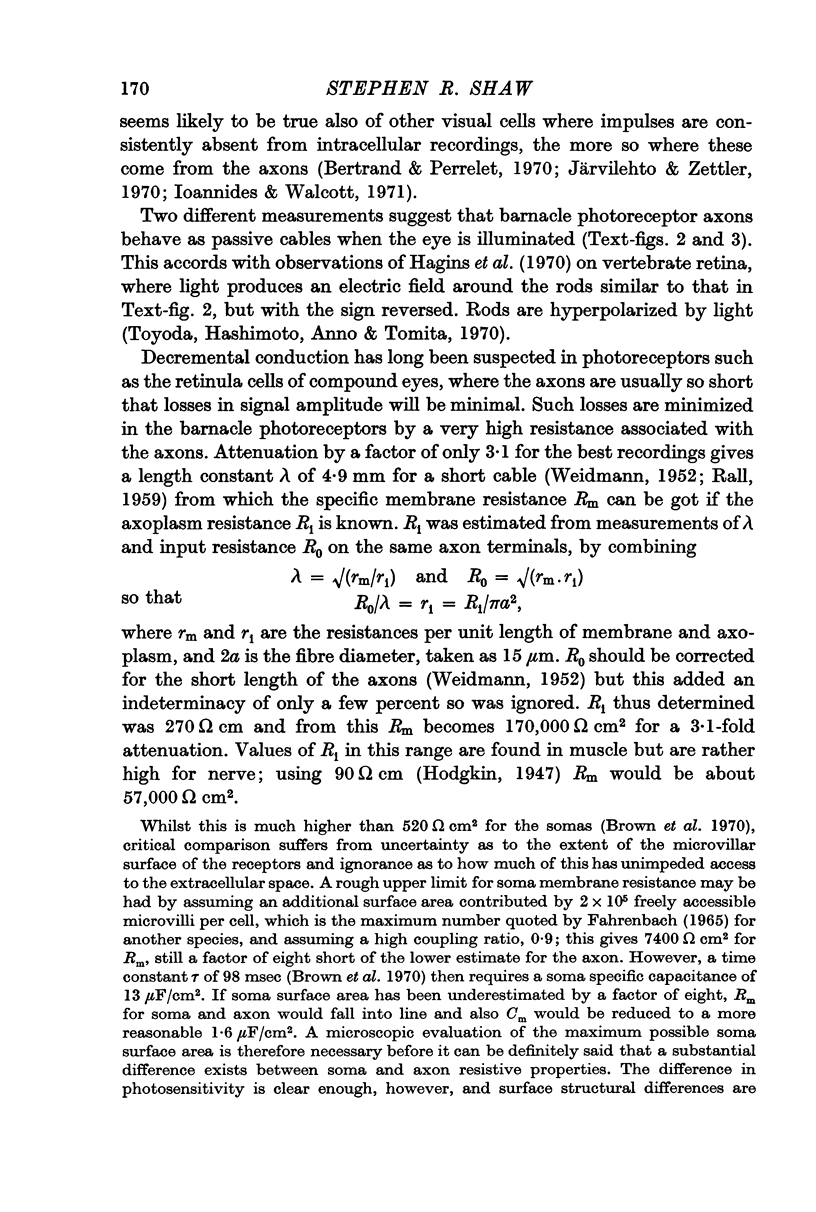
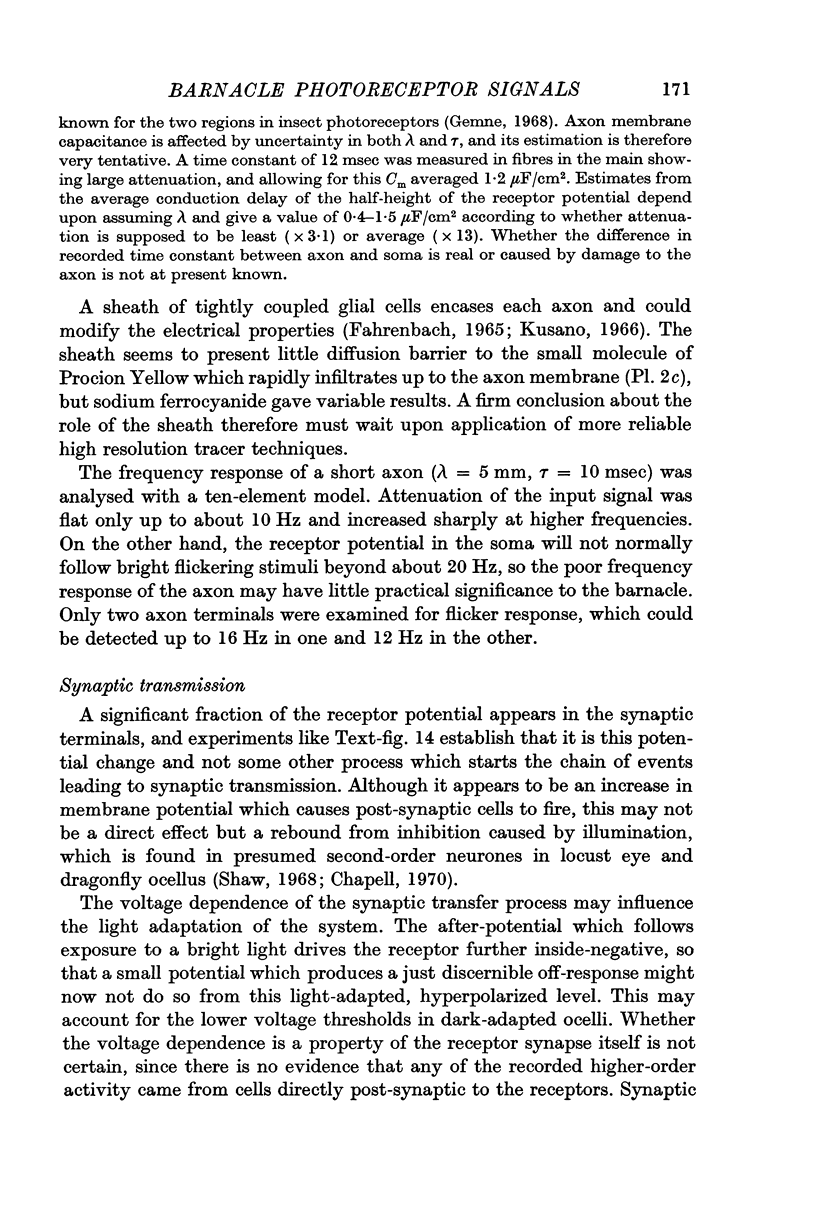
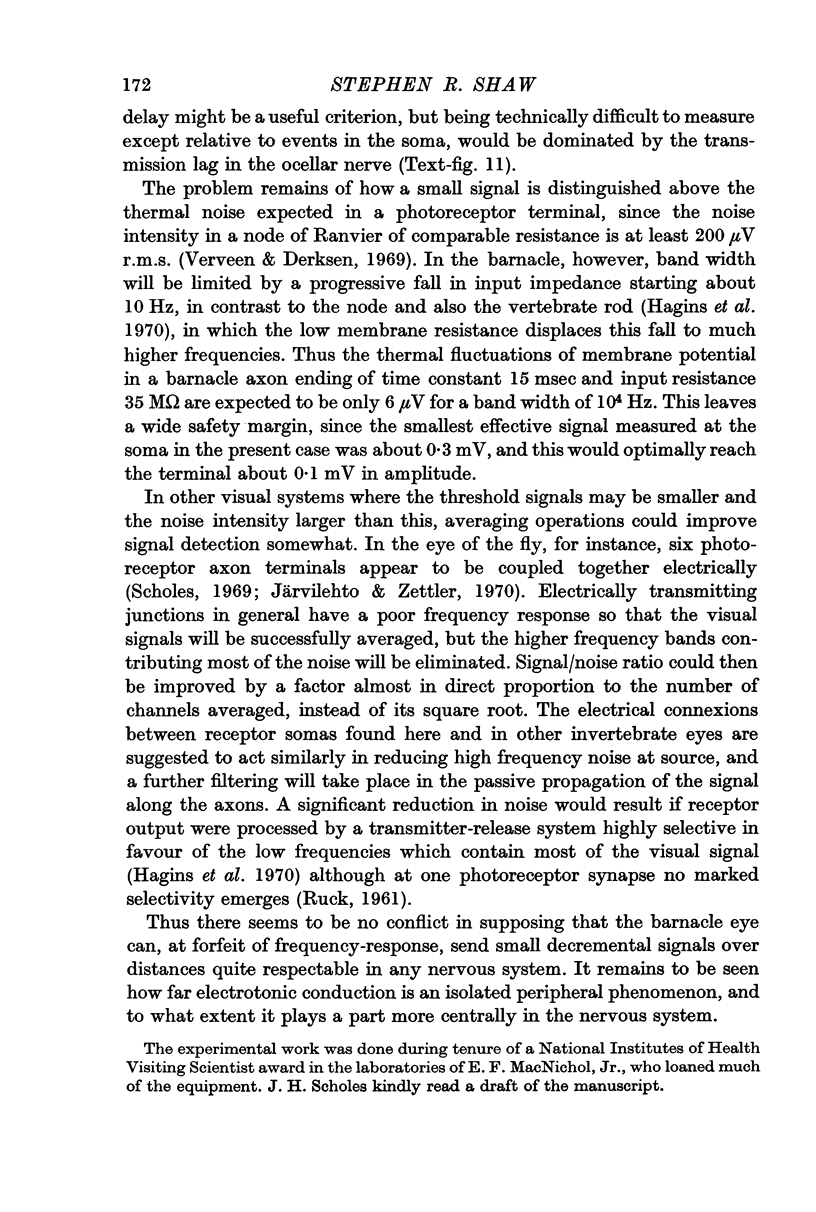
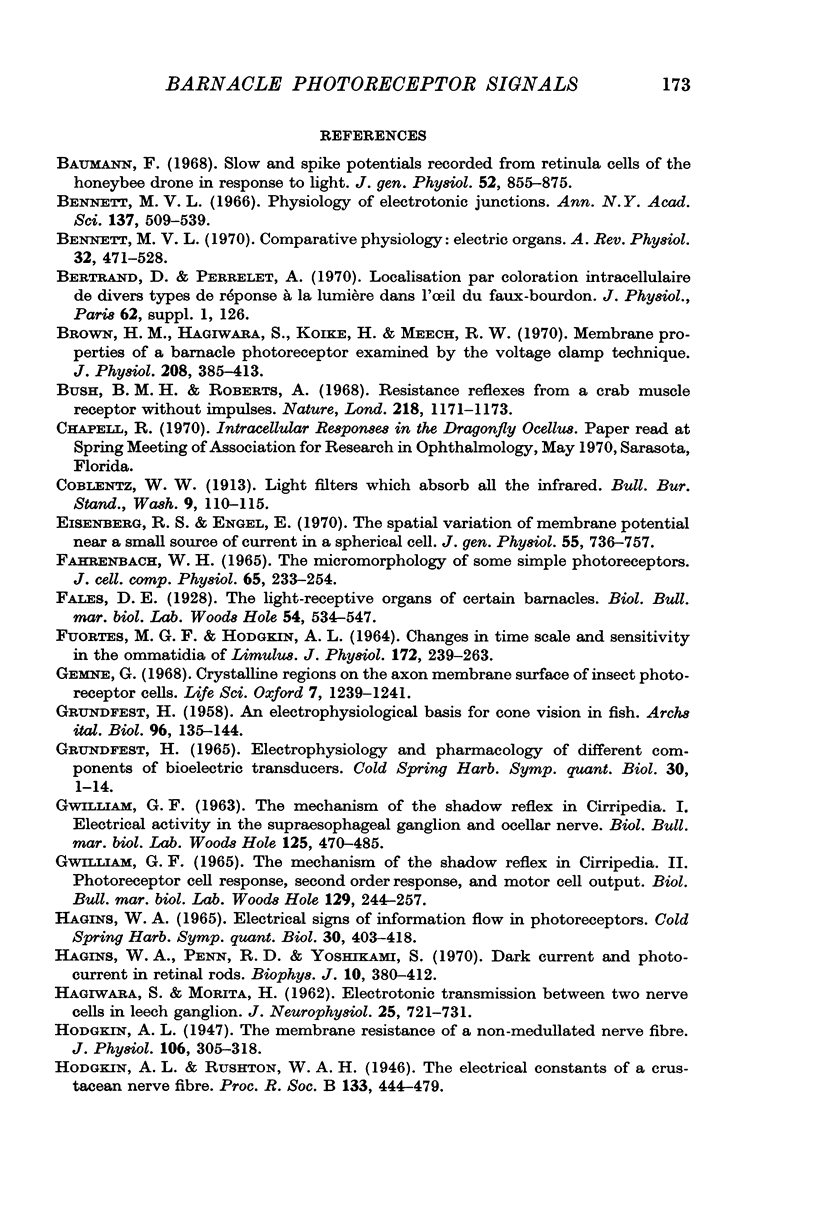
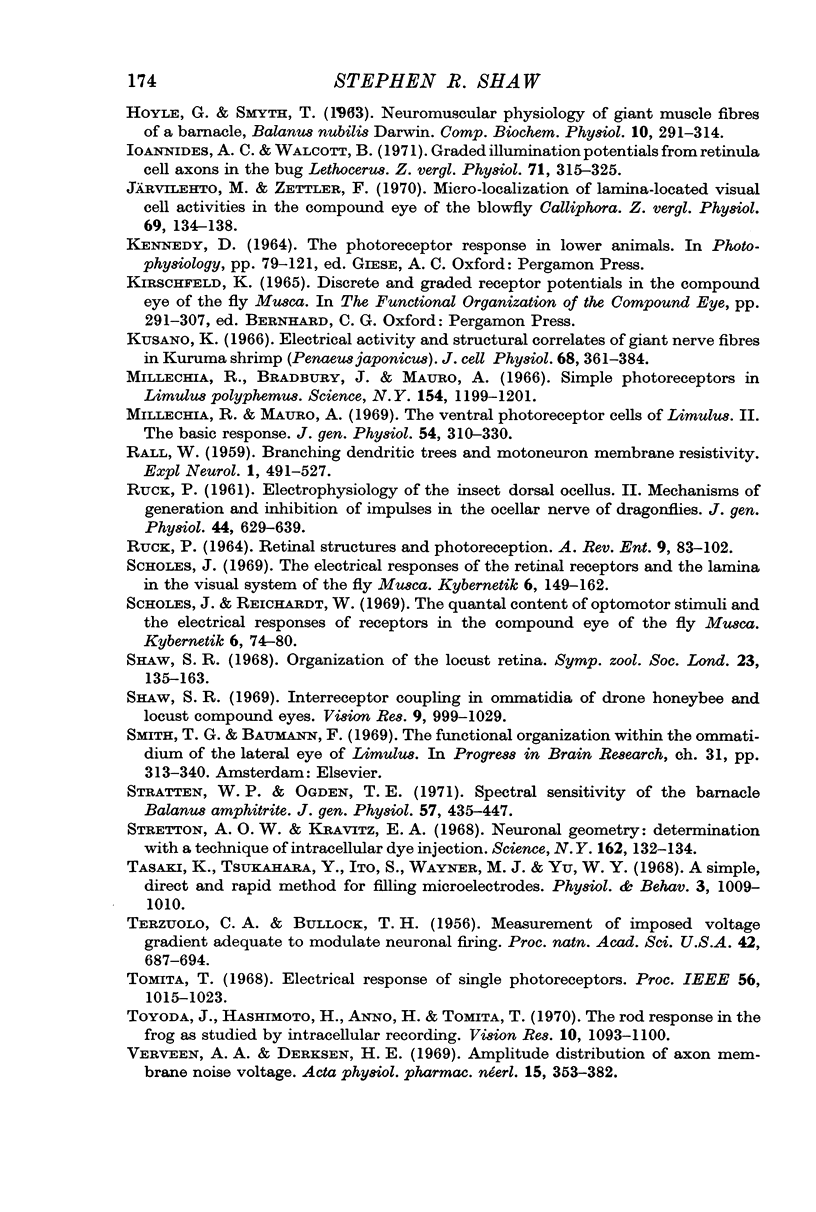
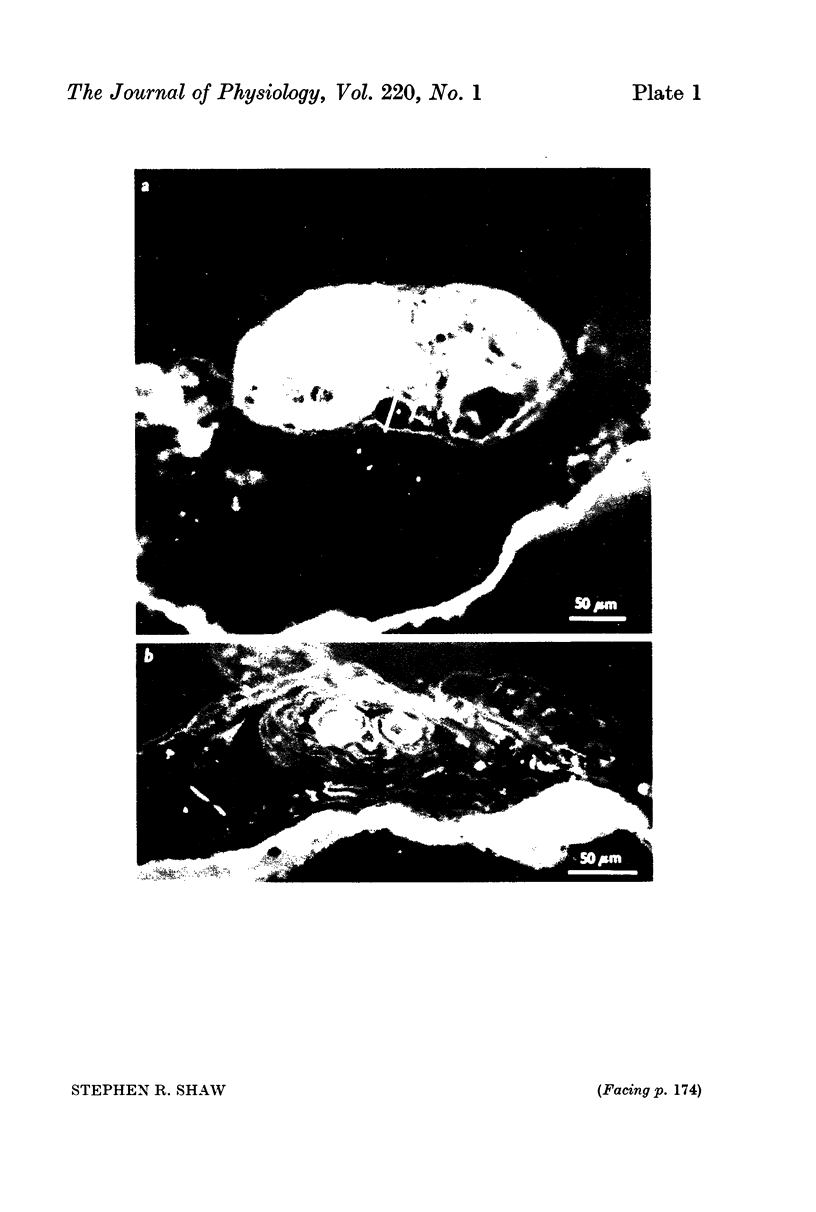
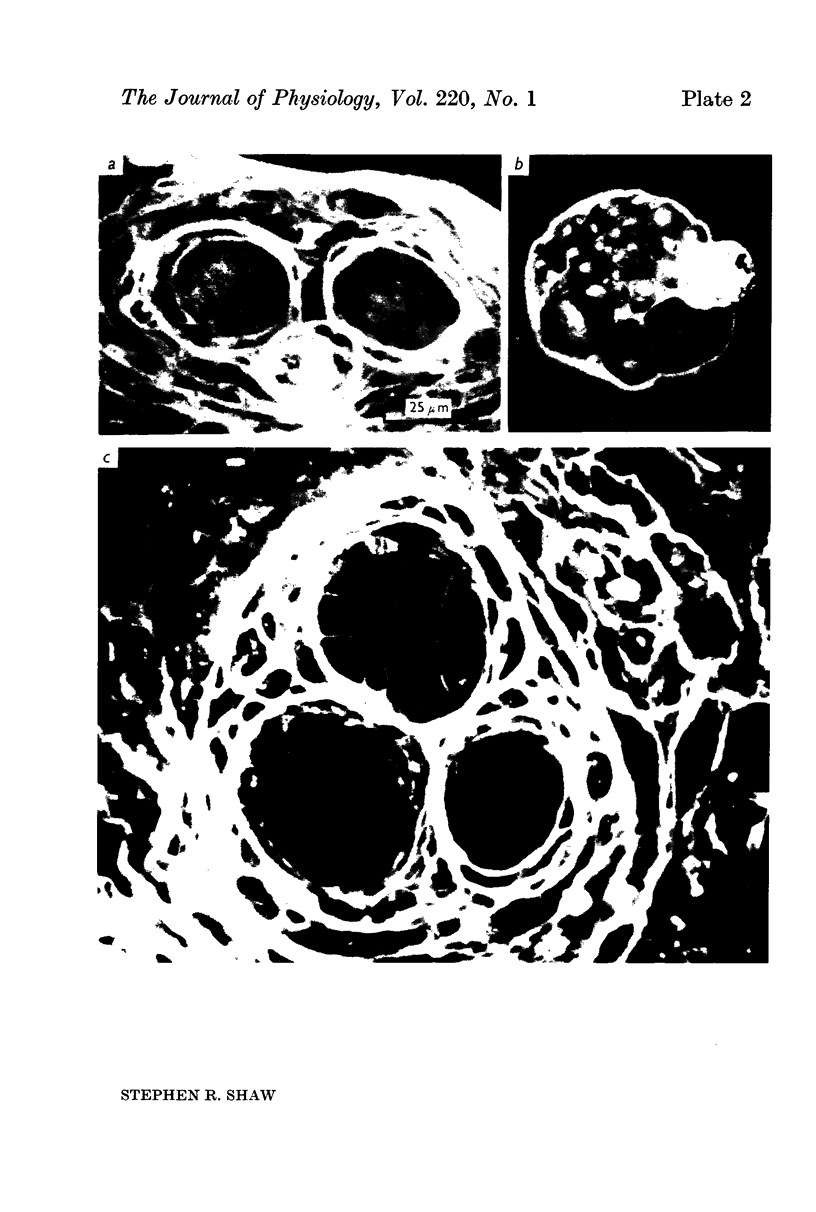
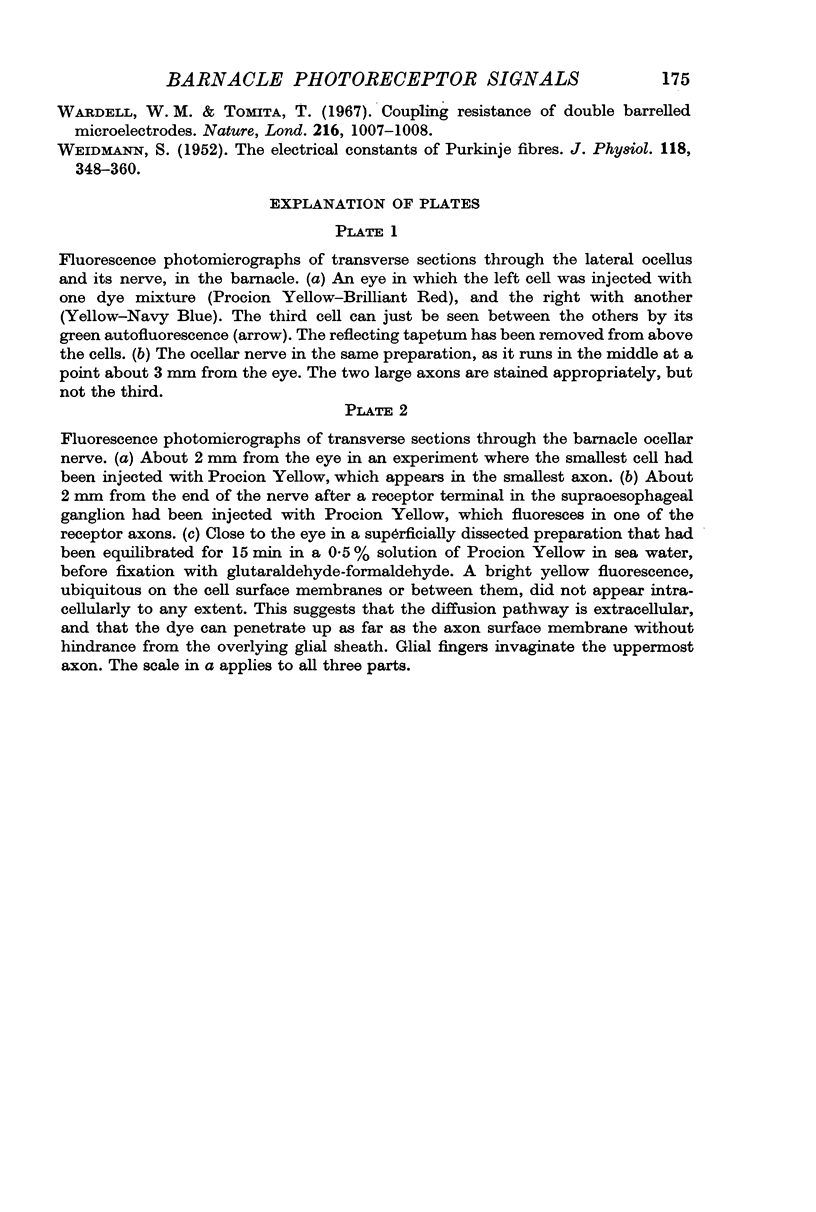
Images in this article
Selected References
These references are in PubMed. This may not be the complete list of references from this article.
- Baumann F. Slow and spike potentials recorded from retinula cells of the honeybee drone in response to light. J Gen Physiol. 1968 Dec;52(6):855–875. doi: 10.1085/jgp.52.6.855. [DOI] [PMC free article] [PubMed] [Google Scholar]
- Bennett M. V. Comparative physiology: electric organs. Annu Rev Physiol. 1970;32:471–528. doi: 10.1146/annurev.ph.32.030170.002351. [DOI] [PubMed] [Google Scholar]
- Bennett M. V. Physiology of electrotonic junctions. Ann N Y Acad Sci. 1966 Jul 14;137(2):509–539. doi: 10.1111/j.1749-6632.1966.tb50178.x. [DOI] [PubMed] [Google Scholar]
- Bertrand D., Perrelet A. Localisation par coloration intracellulaire de divers types de réponse à la lumière dans l'oeil du faux-bourdon. J Physiol (Paris) 1970;62 (Suppl 1):126–127. [PubMed] [Google Scholar]
- Brown H. M., Hagiwara S., Koike H., Meech R. M. Membrane properties of a barnacle photoreceptor examined by the voltage clamp technique. J Physiol. 1970 Jun;208(2):385–413. doi: 10.1113/jphysiol.1970.sp009127. [DOI] [PMC free article] [PubMed] [Google Scholar]
- Bush B. M., Roberts A. Resistance reflexes from a crab muscle receptor without impulses. Nature. 1968 Jun 22;218(5147):1171–1173. doi: 10.1038/2181171a0. [DOI] [PubMed] [Google Scholar]
- Eisenberg R. S., Engel E. The spatial variation of membrane potential near a small source of current in a spherical cell. J Gen Physiol. 1970 Jun;55(6):736–757. doi: 10.1085/jgp.55.6.736. [DOI] [PMC free article] [PubMed] [Google Scholar]
- FUORTES M. G., HODGKIN A. L. CHANGES IN TIME SCALE AND SENSITIVITY IN THE OMMATIDIA OF LIMULUS. J Physiol. 1964 Aug;172:239–263. doi: 10.1113/jphysiol.1964.sp007415. [DOI] [PMC free article] [PubMed] [Google Scholar]
- Gemne G. Crystalline regions on the axon membrane surface of insect photoreceptor cells. Life Sci. 1968 Dec 15;7(24):1239–1241. doi: 10.1016/0024-3205(68)90251-8. [DOI] [PubMed] [Google Scholar]
- Grundfest H. Electrophysiology and pharmacology of different components of bioelectric transducers. Cold Spring Harb Symp Quant Biol. 1965;30:1–14. doi: 10.1101/sqb.1965.030.01.005. [DOI] [PubMed] [Google Scholar]
- HAGIWARA S., MORITA H. Electrotonic transmission between two nerve cells in leech ganglion. J Neurophysiol. 1962 Nov;25:721–731. doi: 10.1152/jn.1962.25.6.721. [DOI] [PubMed] [Google Scholar]
- Hagins W. A. Electrical signs of information flow in photoreceptors. Cold Spring Harb Symp Quant Biol. 1965;30:403–418. doi: 10.1101/sqb.1965.030.01.040. [DOI] [PubMed] [Google Scholar]
- Hagins W. A., Penn R. D., Yoshikami S. Dark current and photocurrent in retinal rods. Biophys J. 1970 May;10(5):380–412. doi: 10.1016/S0006-3495(70)86308-1. [DOI] [PMC free article] [PubMed] [Google Scholar]
- Hodgkin A. L. The membrane resistance of a non-medullated nerve fibre. J Physiol. 1947 Jul 31;106(3):305–318. doi: 10.1113/jphysiol.1947.sp004214. [DOI] [PMC free article] [PubMed] [Google Scholar]
- Millecchia R., Bradbury J., Mauro A. Simple photoreceptors in Limulus polyphemus. Science. 1966 Dec 2;154(3753):1199–1201. doi: 10.1126/science.154.3753.1199. [DOI] [PubMed] [Google Scholar]
- Millecchia R., Mauro A. The ventral photoreceptor cells of Limulus. II. The basic photoresponse. J Gen Physiol. 1969 Sep;54(3):310–330. doi: 10.1085/jgp.54.3.310. [DOI] [PMC free article] [PubMed] [Google Scholar]
- RALL W. Branching dendritic trees and motoneuron membrane resistivity. Exp Neurol. 1959 Nov;1:491–527. doi: 10.1016/0014-4886(59)90046-9. [DOI] [PubMed] [Google Scholar]
- RUCK P. Electrophysiology of the insect dorsal ocellus. II. Mechanisms of generation and inhibition of impulses in the ocellar nerve of dragonflies. J Gen Physiol. 1961 Jan;44:629–639. doi: 10.1085/jgp.44.3.629. [DOI] [PMC free article] [PubMed] [Google Scholar]
- Scholes J., Reichardt W. The quantal content of optomotor stimuli and the electrical responses of receptors in the compound eye of the fly Musca. Kybernetik. 1969 Jun;6(2):74–79. doi: 10.1007/BF00276908. [DOI] [PubMed] [Google Scholar]
- Scholes J. The electrical responses of the retinal receptors and the lamina in the visual system of the fly Musca. Kybernetik. 1969 Sep;6(4):149–162. doi: 10.1007/BF00274109. [DOI] [PubMed] [Google Scholar]
- Shaw S. R. Interreceptor coupling in ommatidia of drone honeybee and locust compound eyes. Vision Res. 1969 Sep;9(9):999–1029. doi: 10.1016/0042-6989(69)90044-3. [DOI] [PubMed] [Google Scholar]
- Smith T. G., Baumann F. The functional organization within the ommatidium of the lateral eye of limulus. Prog Brain Res. 1969;31:313–349. doi: 10.1016/S0079-6123(08)63248-3. [DOI] [PubMed] [Google Scholar]
- Stratten W. P., Ogden T. E. Spectral sensitivity of the barnacle, Balanus amphitrite. J Gen Physiol. 1971 Apr;57(4):435–447. doi: 10.1085/jgp.57.4.435. [DOI] [PMC free article] [PubMed] [Google Scholar]
- Stretton A. O., Kravitz E. A. Neuronal geometry: determination with a technique of intracellular dye injection. Science. 1968 Oct 4;162(3849):132–134. doi: 10.1126/science.162.3849.132. [DOI] [PubMed] [Google Scholar]
- Terzuolo C. A., Bullock T. H. MEASUREMENT OF IMPOSED VOLTAGE GRADIENT ADEQUATE TO MODULATE NEURONAL FIRING. Proc Natl Acad Sci U S A. 1956 Sep;42(9):687–694. doi: 10.1073/pnas.42.9.687. [DOI] [PMC free article] [PubMed] [Google Scholar]
- Toyoda J., Hashimoto H., Anno H., Tomita T. The rod response in the frog and studies by intracellular recording. Vision Res. 1970 Nov;10(11):1093–1100. doi: 10.1016/0042-6989(70)90026-x. [DOI] [PubMed] [Google Scholar]
- Verveen A. A., Derksen H. E. Amplitude distribution of axon membrane noise voltage. Acta Physiol Pharmacol Neerl. 1969 Aug;15(3):353–379. [PubMed] [Google Scholar]
- WEIDMANN S. The electrical constants of Purkinje fibres. J Physiol. 1952 Nov;118(3):348–360. doi: 10.1113/jphysiol.1952.sp004799. [DOI] [PMC free article] [PubMed] [Google Scholar]
- Wardell W. M., Tomita T. Coupling resistance of double barrelled microelectrodes. Nature. 1967 Dec 9;216(5119):1007–1008. doi: 10.1038/2161007a0. [DOI] [PubMed] [Google Scholar]