Abstract
1. The whole cell variant of the patch clamp technique was used to record high voltage-activated Ca2+ currents and Ca(2+)-activated Cl- tail currents from cultured neonatal rat dorsal root ganglion neurones. The aim of the project was to use these currents as physiological indices of intracellular Ca2+ regulation under control conditions and in the presence of metabolic inhibitors. 2. Carbonyl cyanide p-trifluoromethoxyphenylhydrazone (5 microM) and sodium cyanide (1 microM) inhibited Ca2+ currents within 20 s, even when ATP was present in the patch pipette solution, suggesting a direct action on Ca2+ channels. These metabolic inhibitors did not affect Ca2+ current 'run down' or inactivation kinetics. 3. Cultured neonatal dorsal root ganglion neurones of the rat were relatively insensitive to the removal of glucose and ATP from the recording solutions for up to 3 h. These data suggest that the Ca2+ homeostatic mechanisms in these cells are highly resistant to metabolic insult. 4. However 2-deoxy-D-glucose (5 mM) in the extracellular recording medium with no ATP or glucose present did prolong the deactivation time of Ca(2+)-activated Cl- tail currents and increase the total charge flow following activation of a 500 ms voltage-activated Ca2+ current. This effect was prevented by inclusion of D-fructose 1,6-diphosphate (500 microM) in the patch pipette solution. 5. We conclude that some agents used to induce chemical hypoxia, such as carbonyl cyanide p-trifluoromethoxyphenylhydrazone and sodium cyanide, may interact directly with voltage-activated Ca2+ channels and are therefore not appropriate for use in studying disturbed neuronal Ca2+ homeostasis. However, the use of 2-deoxy-D-glucose in the absence of glucose and ATP does represent a model of disturbed Ca2+ homeostasis in cultured dorsal root ganglion neurones. In this study we have combined the whole cell recording technique with cultured neurones under conditions which produce a degree of metabolic stress as reflected by prolonged Ca(2+)-activated Cl- tail currents. The reduced efficiency of handling of intracellular Ca2+ loads may be an important factor contributing to the onset of neuronal damage during hypoxia and ischaemia.
Full text
PDF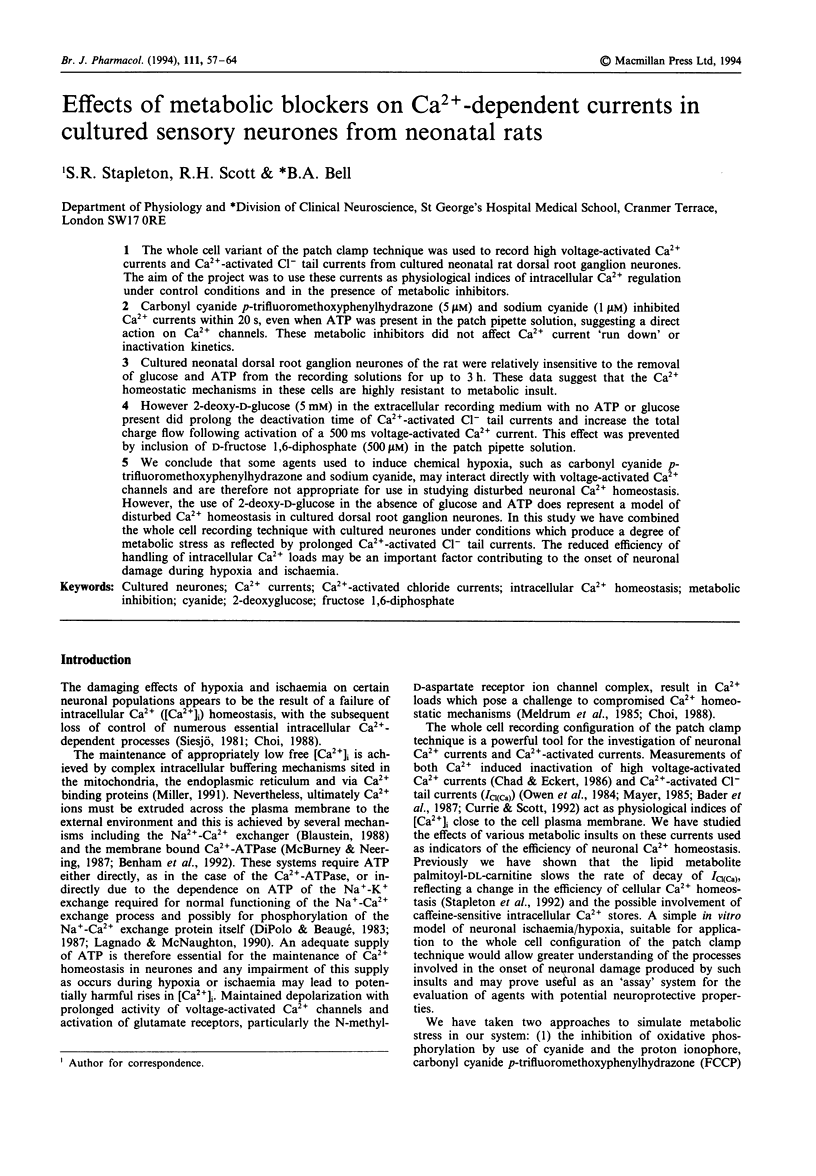
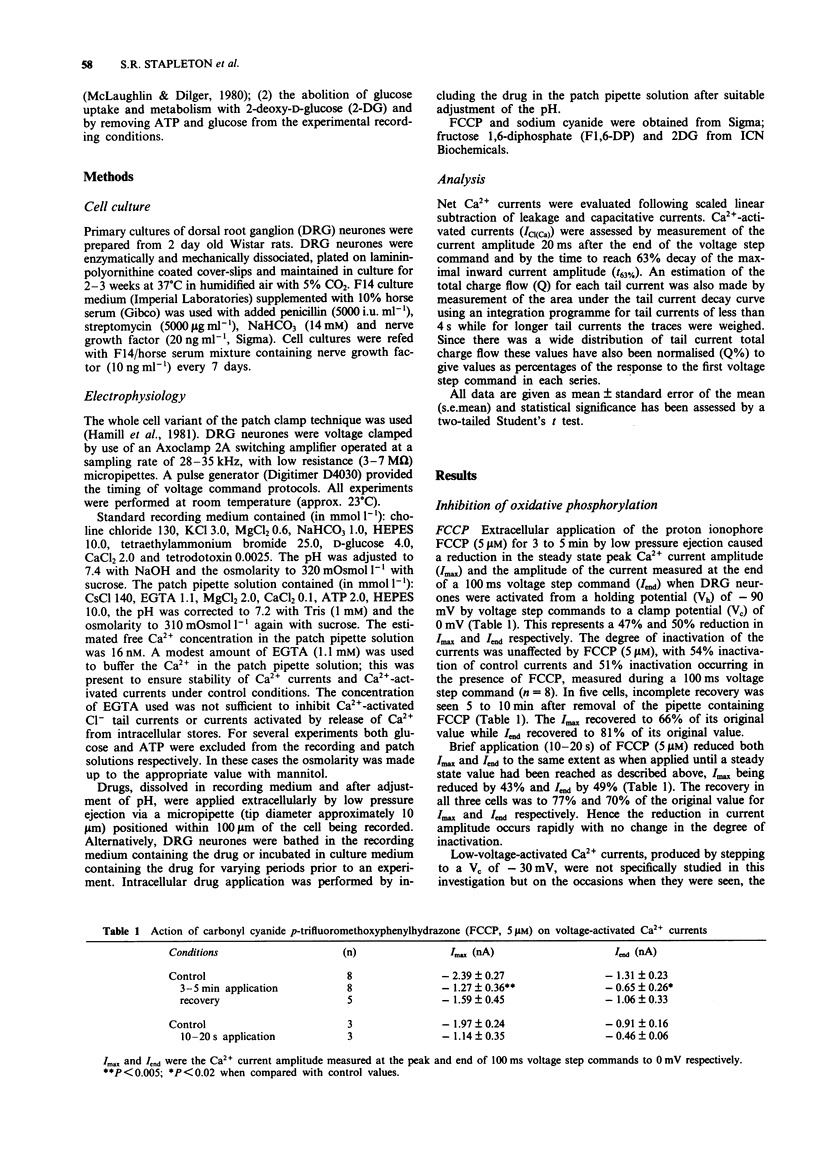
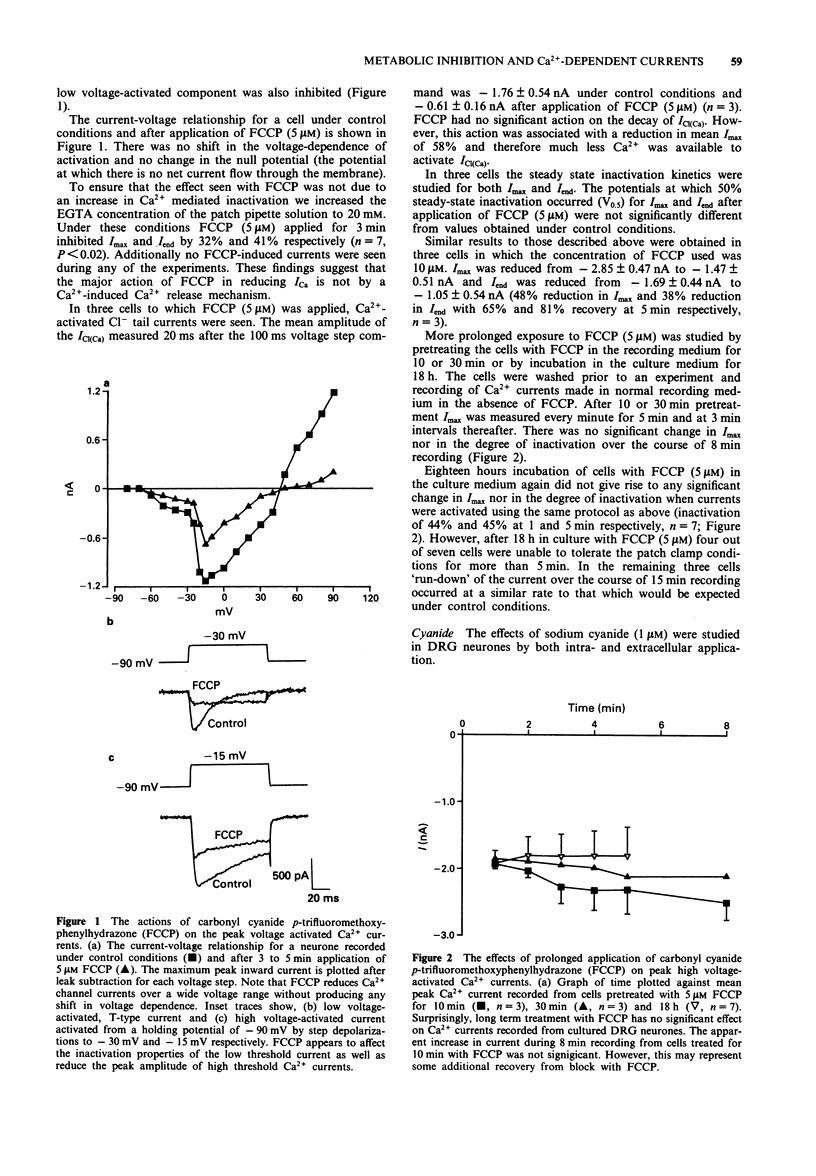
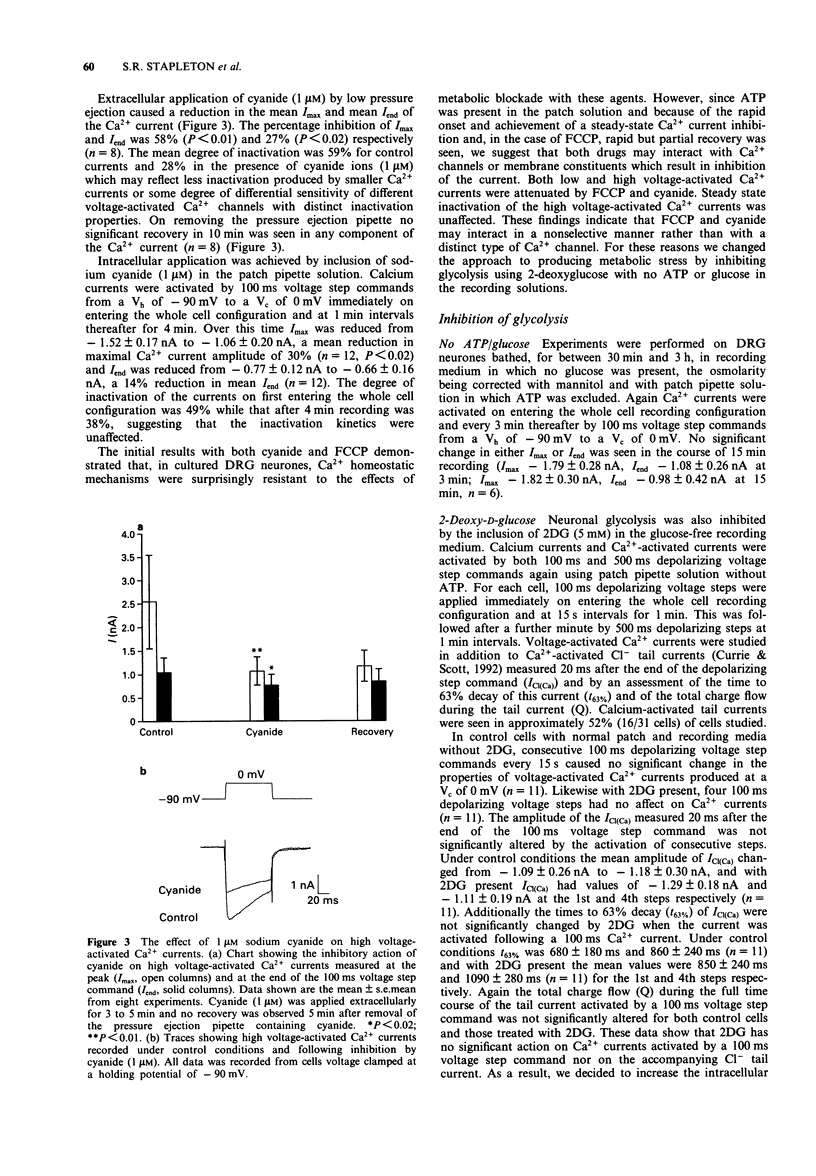
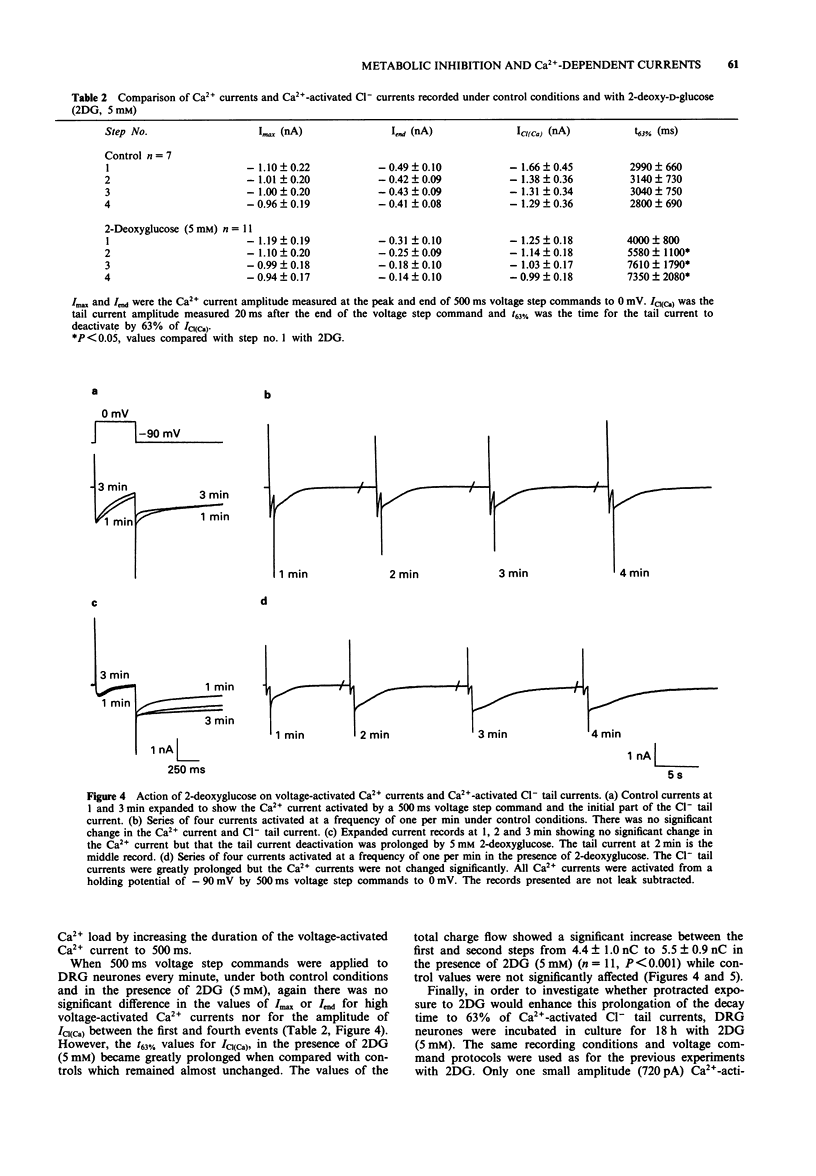
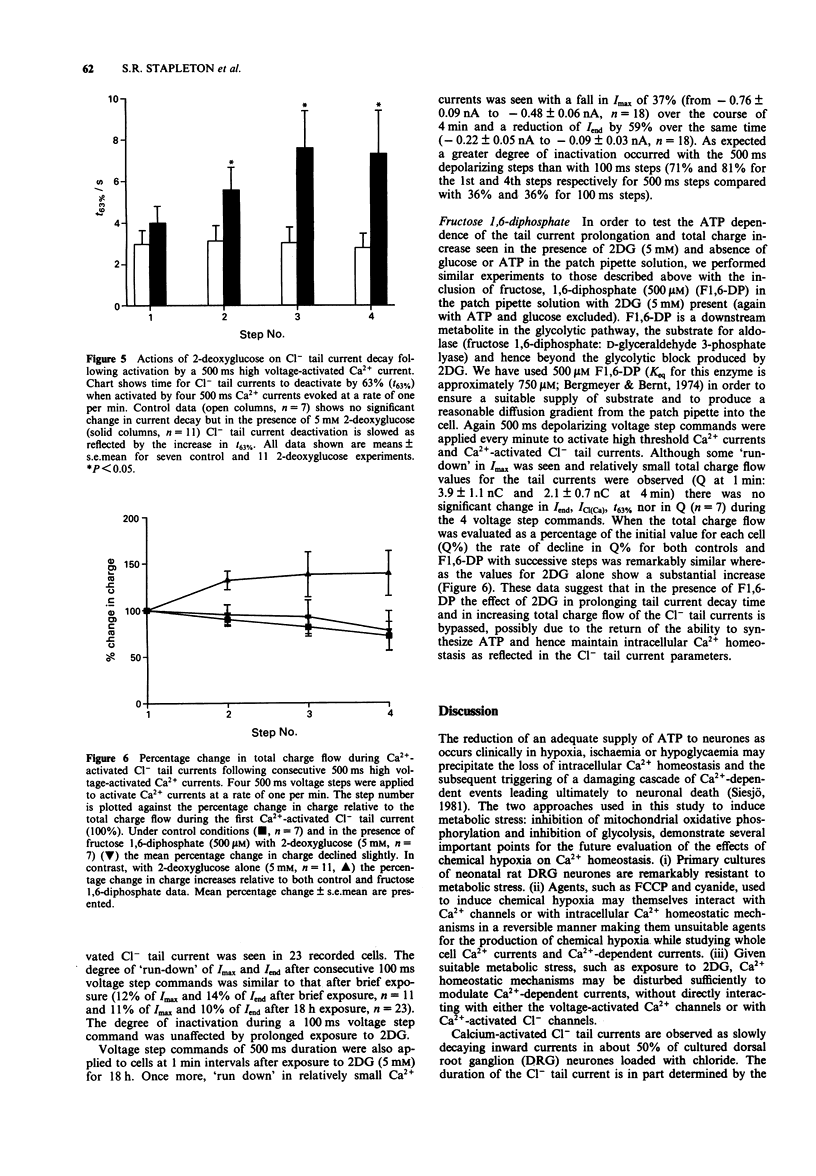
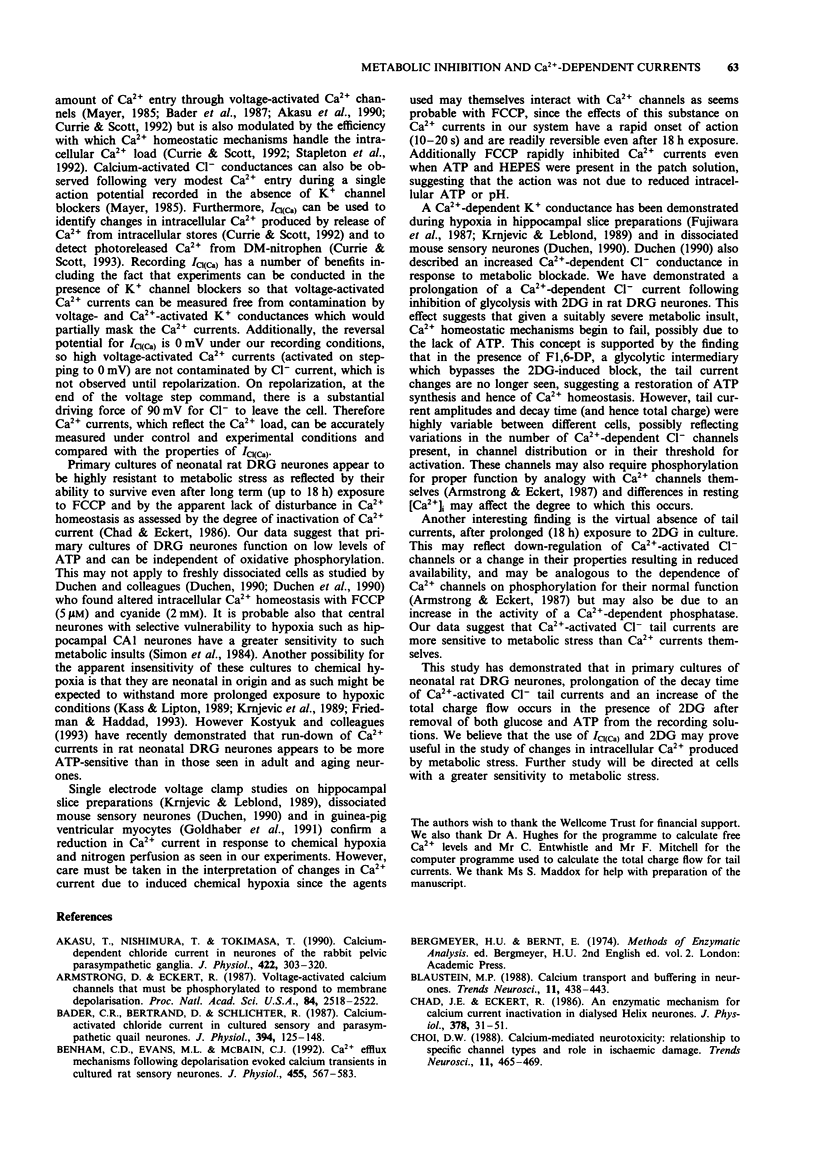
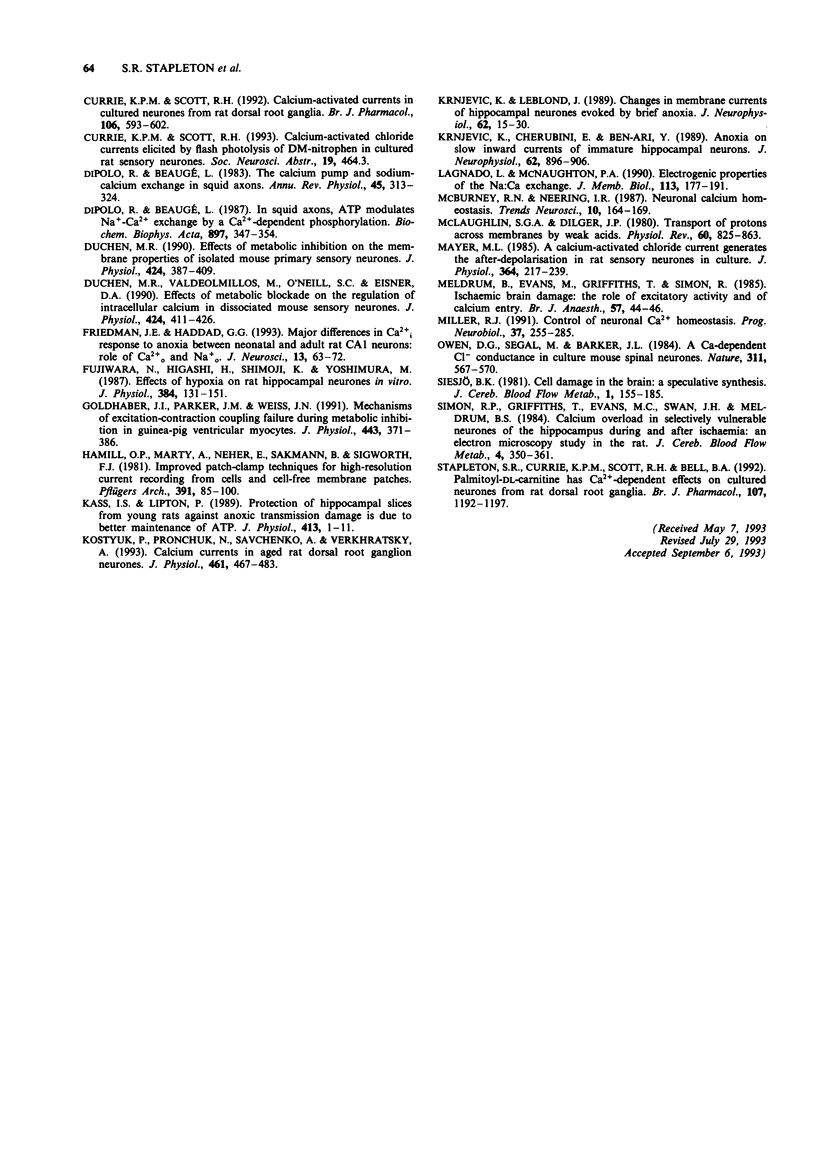
Selected References
These references are in PubMed. This may not be the complete list of references from this article.
- Akasu T., Nishimura T., Tokimasa T. Calcium-dependent chloride current in neurones of the rabbit pelvic parasympathetic ganglia. J Physiol. 1990 Mar;422:303–320. doi: 10.1113/jphysiol.1990.sp017985. [DOI] [PMC free article] [PubMed] [Google Scholar]
- Armstrong D., Eckert R. Voltage-activated calcium channels that must be phosphorylated to respond to membrane depolarization. Proc Natl Acad Sci U S A. 1987 Apr;84(8):2518–2522. doi: 10.1073/pnas.84.8.2518. [DOI] [PMC free article] [PubMed] [Google Scholar]
- Bader C. R., Bertrand D., Schlichter R. Calcium-activated chloride current in cultured sensory and parasympathetic quail neurones. J Physiol. 1987 Dec;394:125–148. doi: 10.1113/jphysiol.1987.sp016863. [DOI] [PMC free article] [PubMed] [Google Scholar]
- Benham C. D., Evans M. L., McBain C. J. Ca2+ efflux mechanisms following depolarization evoked calcium transients in cultured rat sensory neurones. J Physiol. 1992 Sep;455:567–583. doi: 10.1113/jphysiol.1992.sp019316. [DOI] [PMC free article] [PubMed] [Google Scholar]
- Blaustein M. P. Calcium transport and buffering in neurons. Trends Neurosci. 1988 Oct;11(10):438–443. doi: 10.1016/0166-2236(88)90195-6. [DOI] [PubMed] [Google Scholar]
- Chad J. E., Eckert R. An enzymatic mechanism for calcium current inactivation in dialysed Helix neurones. J Physiol. 1986 Sep;378:31–51. doi: 10.1113/jphysiol.1986.sp016206. [DOI] [PMC free article] [PubMed] [Google Scholar]
- Choi D. W. Calcium-mediated neurotoxicity: relationship to specific channel types and role in ischemic damage. Trends Neurosci. 1988 Oct;11(10):465–469. doi: 10.1016/0166-2236(88)90200-7. [DOI] [PubMed] [Google Scholar]
- Currie K. P., Scott R. H. Calcium-activated currents in cultured neurones from rat dorsal root ganglia. Br J Pharmacol. 1992 Jul;106(3):593–602. doi: 10.1111/j.1476-5381.1992.tb14381.x. [DOI] [PMC free article] [PubMed] [Google Scholar]
- DiPolo R., Beaugé L. In squid axons, ATP modulates Na+-Ca2+ exchange by a Ca2+i-dependent phosphorylation. Biochim Biophys Acta. 1987 Mar 12;897(3):347–354. doi: 10.1016/0005-2736(87)90432-9. [DOI] [PubMed] [Google Scholar]
- DiPolo R., Beaugé L. The calcium pump and sodium-calcium exchange in squid axons. Annu Rev Physiol. 1983;45:313–324. doi: 10.1146/annurev.ph.45.030183.001525. [DOI] [PubMed] [Google Scholar]
- Duchen M. R. Effects of metabolic inhibition on the membrane properties of isolated mouse primary sensory neurones. J Physiol. 1990 May;424:387–409. doi: 10.1113/jphysiol.1990.sp018073. [DOI] [PMC free article] [PubMed] [Google Scholar]
- Duchen M. R., Valdeolmillos M., O'Neill S. C., Eisner D. A. Effects of metabolic blockade on the regulation of intracellular calcium in dissociated mouse sensory neurones. J Physiol. 1990 May;424:411–426. doi: 10.1113/jphysiol.1990.sp018074. [DOI] [PMC free article] [PubMed] [Google Scholar]
- Friedman J. E., Haddad G. G. Major differences in Ca2+i response to anoxia between neonatal and adult rat CA1 neurons: role of Ca2+o and Na+o. J Neurosci. 1993 Jan;13(1):63–72. doi: 10.1523/JNEUROSCI.13-01-00063.1993. [DOI] [PMC free article] [PubMed] [Google Scholar]
- Fujiwara N., Higashi H., Shimoji K., Yoshimura M. Effects of hypoxia on rat hippocampal neurones in vitro. J Physiol. 1987 Mar;384:131–151. doi: 10.1113/jphysiol.1987.sp016447. [DOI] [PMC free article] [PubMed] [Google Scholar]
- Goldhaber J. I., Parker J. M., Weiss J. N. Mechanisms of excitation-contraction coupling failure during metabolic inhibition in guinea-pig ventricular myocytes. J Physiol. 1991 Nov;443:371–386. doi: 10.1113/jphysiol.1991.sp018838. [DOI] [PMC free article] [PubMed] [Google Scholar]
- Hamill O. P., Marty A., Neher E., Sakmann B., Sigworth F. J. Improved patch-clamp techniques for high-resolution current recording from cells and cell-free membrane patches. Pflugers Arch. 1981 Aug;391(2):85–100. doi: 10.1007/BF00656997. [DOI] [PubMed] [Google Scholar]
- Kass I. S., Lipton P. Protection of hippocampal slices from young rats against anoxic transmission damage is due to better maintenance of ATP. J Physiol. 1989 Jun;413:1–11. doi: 10.1113/jphysiol.1989.sp017638. [DOI] [PMC free article] [PubMed] [Google Scholar]
- Kostyuk P., Pronchuk N., Savchenko A., Verkhratsky A. Calcium currents in aged rat dorsal root ganglion neurones. J Physiol. 1993 Feb;461:467–483. doi: 10.1113/jphysiol.1993.sp019523. [DOI] [PMC free article] [PubMed] [Google Scholar]
- Krnjević K., Cherubini E., Ben-Ari Y. Anoxia on slow inward currents of immature hippocampal neurons. J Neurophysiol. 1989 Oct;62(4):896–906. doi: 10.1152/jn.1989.62.4.896. [DOI] [PubMed] [Google Scholar]
- Krnjević K., Leblond J. Changes in membrane currents of hippocampal neurons evoked by brief anoxia. J Neurophysiol. 1989 Jul;62(1):15–30. doi: 10.1152/jn.1989.62.1.15. [DOI] [PubMed] [Google Scholar]
- Lagnado L., McNaughton P. A. Electrogenic properties of the Na:Ca exchange. J Membr Biol. 1990 Feb;113(3):177–191. doi: 10.1007/BF01870070. [DOI] [PubMed] [Google Scholar]
- Mayer M. L. A calcium-activated chloride current generates the after-depolarization of rat sensory neurones in culture. J Physiol. 1985 Jul;364:217–239. doi: 10.1113/jphysiol.1985.sp015740. [DOI] [PMC free article] [PubMed] [Google Scholar]
- McLaughlin S. G., Dilger J. P. Transport of protons across membranes by weak acids. Physiol Rev. 1980 Jul;60(3):825–863. doi: 10.1152/physrev.1980.60.3.825. [DOI] [PubMed] [Google Scholar]
- Meldrum B., Evans M., Griffiths T., Simon R. Ischaemic brain damage: the role of excitatory activity and of calcium entry. Br J Anaesth. 1985 Jan;57(1):44–46. doi: 10.1093/bja/57.1.44. [DOI] [PubMed] [Google Scholar]
- Miller R. J. The control of neuronal Ca2+ homeostasis. Prog Neurobiol. 1991;37(3):255–285. doi: 10.1016/0301-0082(91)90028-y. [DOI] [PubMed] [Google Scholar]
- Owen D. G., Segal M., Barker J. L. A Ca-dependent Cl- conductance in cultured mouse spinal neurones. Nature. 1984 Oct 11;311(5986):567–570. doi: 10.1038/311567a0. [DOI] [PubMed] [Google Scholar]
- Siesjö B. K. Cell damage in the brain: a speculative synthesis. J Cereb Blood Flow Metab. 1981;1(2):155–185. doi: 10.1038/jcbfm.1981.18. [DOI] [PubMed] [Google Scholar]
- Simon R. P., Griffiths T., Evans M. C., Swan J. H., Meldrum B. S. Calcium overload in selectively vulnerable neurons of the hippocampus during and after ischemia: an electron microscopy study in the rat. J Cereb Blood Flow Metab. 1984 Sep;4(3):350–361. doi: 10.1038/jcbfm.1984.52. [DOI] [PubMed] [Google Scholar]
- Stapleton S. R., Currie K. P., Scott R. H., Bell B. A. Palmitoyl-DL-carnitine has calcium-dependent effects on cultured neurones from rat dorsal root ganglia. Br J Pharmacol. 1992 Dec;107(4):1192–1197. doi: 10.1111/j.1476-5381.1992.tb13427.x. [DOI] [PMC free article] [PubMed] [Google Scholar]