Abstract
The hallmark of ischemic acute renal failure is a rapid and early decline in proximal tubule ATP. Since we have previously shown that over half of apical microfilament losses occur within the first 5 min of experimental ischemic injury, we postulated that microfilament (F-actin) structure and cellular location are dependent on cellular ATP levels. To test this hypothesis, we used maleic acid to selectively inhibit renal cortical ATP production in vivo. Maleic acid significantly decreased tissue ATP and apical F-actin in a dose-dependent manner relative to equimolar sodium chloride controls, yet higher doses of maleic acid quantitatively resulted in net actin polymerization, primarily in the cytoplasm. Functionally, maleic acid decreased glomerular filtration rate (GFR) and tubular reabsorption of sodium (TRNa) in a dose-dependent manner relative to sodium chloride controls. Administration of exogenous ATP resulted in significant increases in tissue ATP, net actin depolymerization, and relocation of F-actin from the cytoplasm back to the apical surface coinciding with increases in GFR and TRNa. Thus, ATP depletion induced by maleic acid resulted in significant cytoskeletal and functional alterations that were ameliorated by exogenous ATP. We therefore conclude that the structure and cellular location of F-actin necessary for normal functioning of proximal tubule cells in vivo is dependent on tissue ATP levels.
Full text
PDF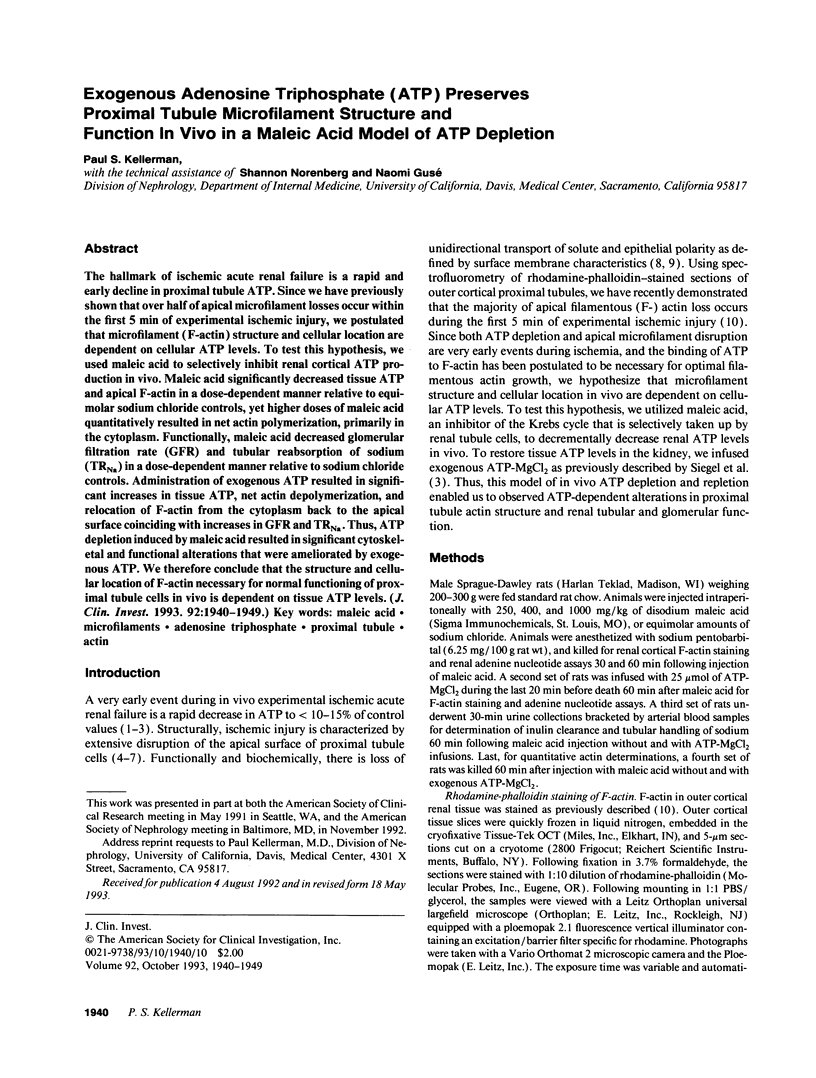
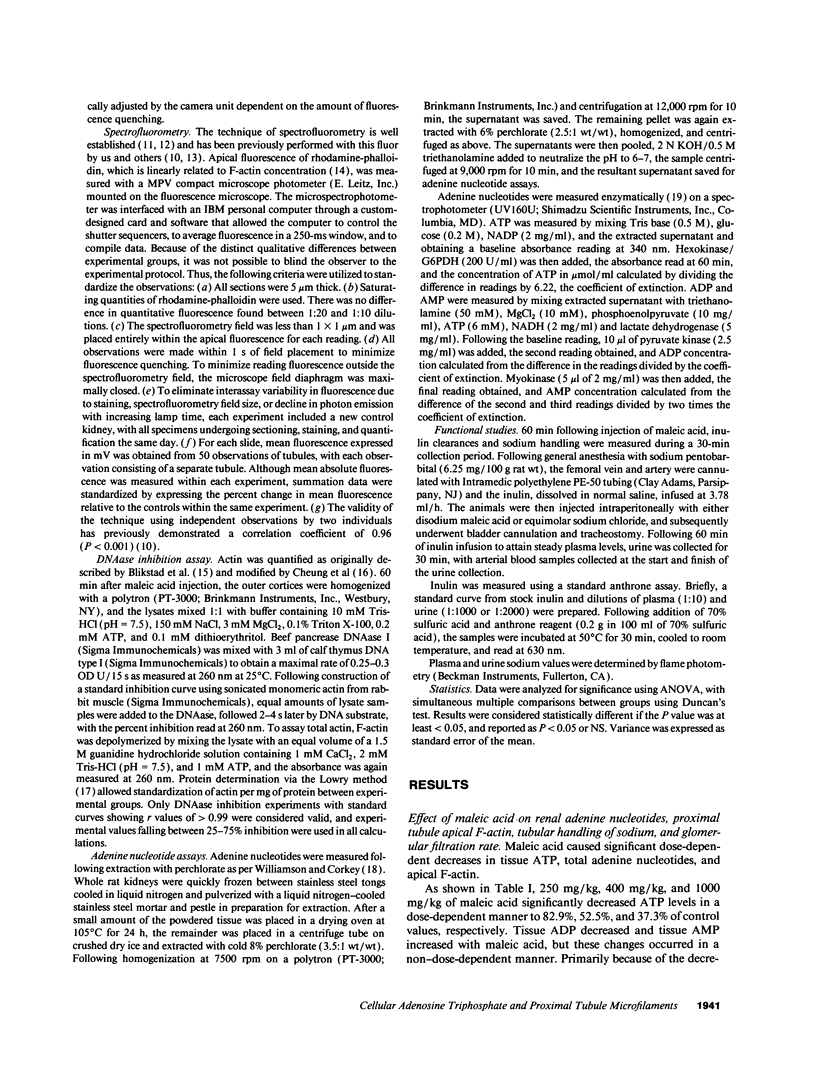
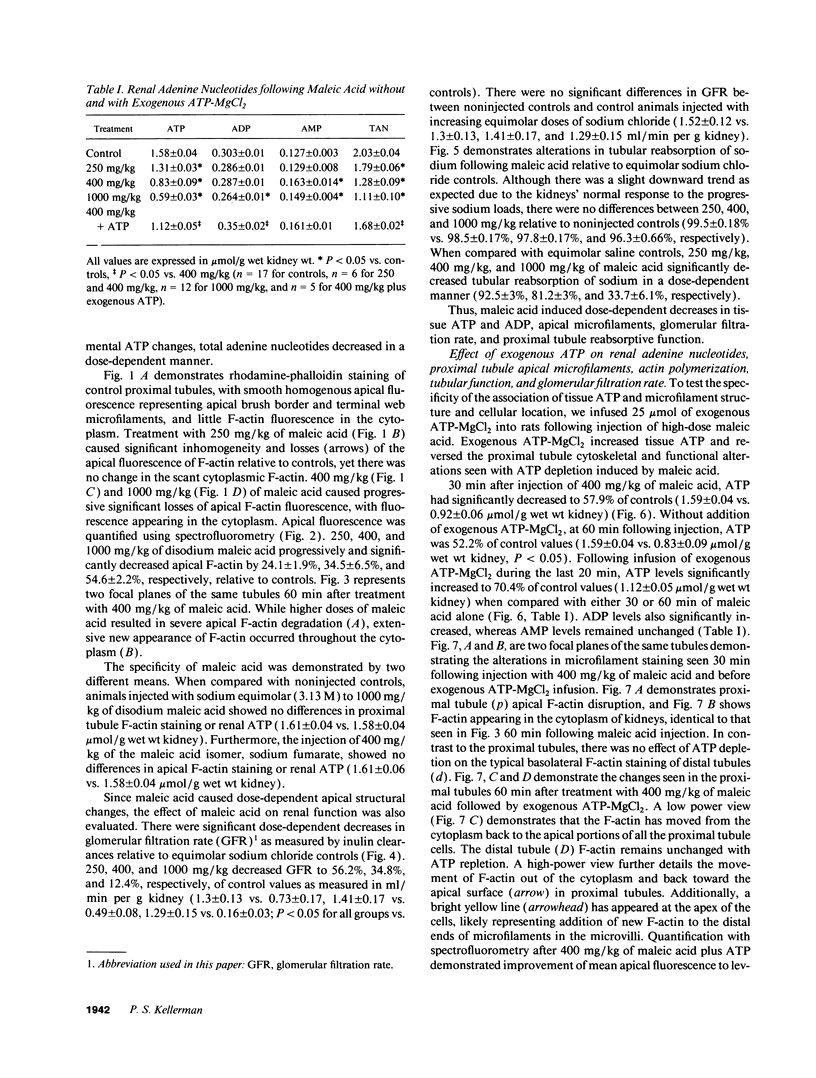
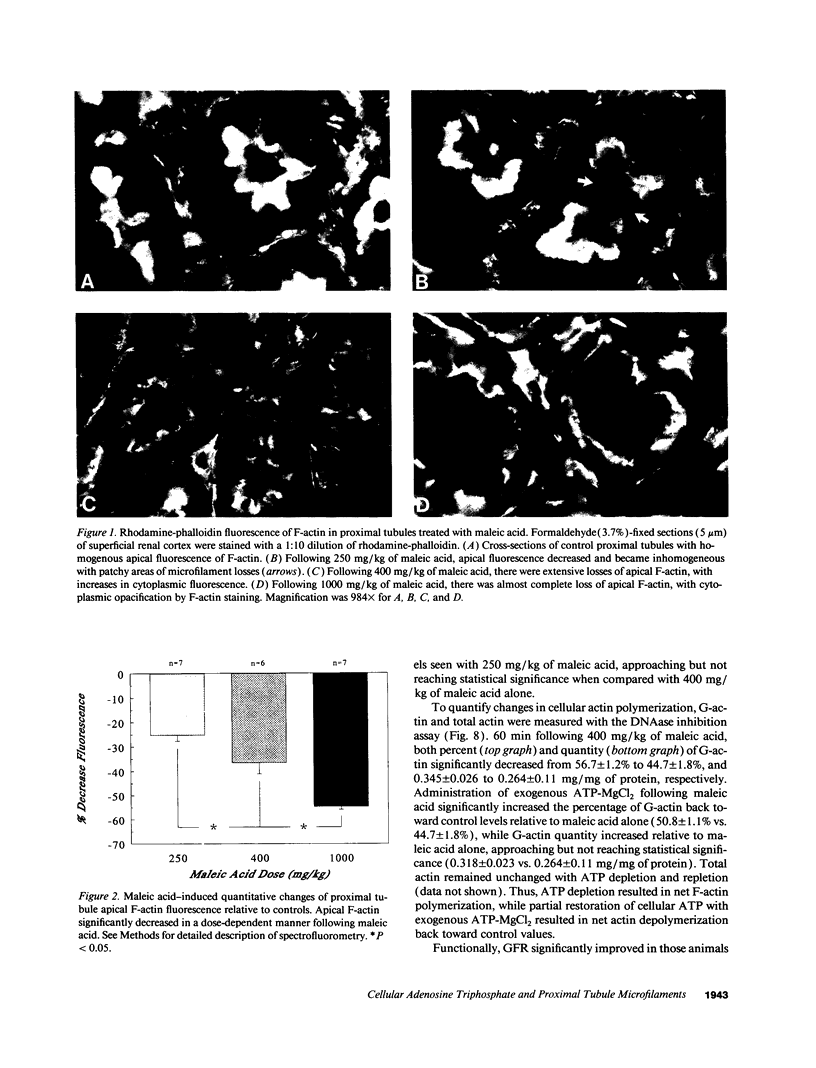
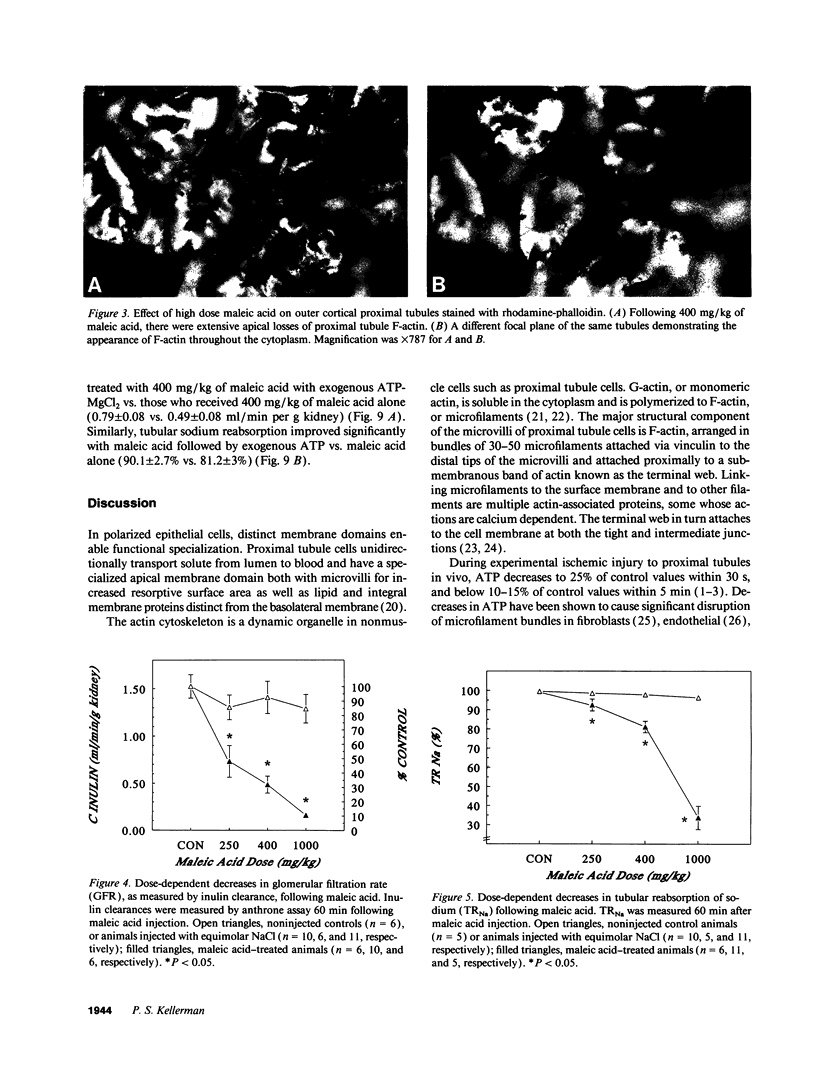
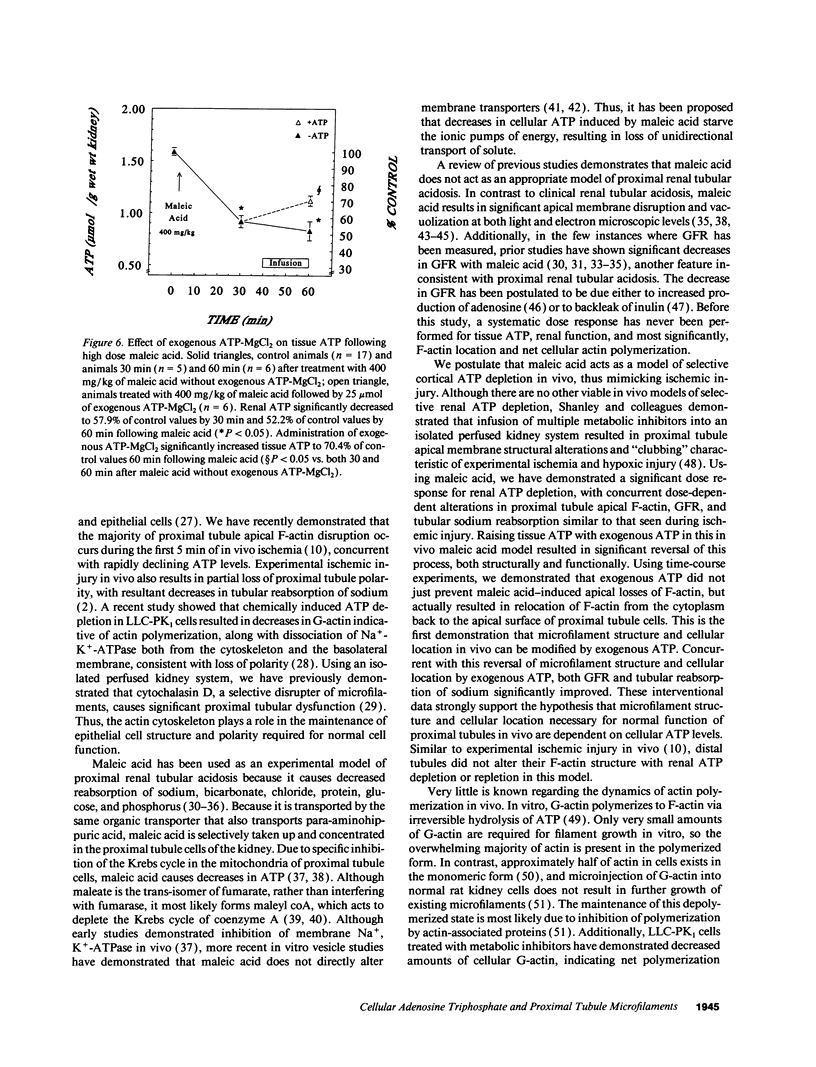
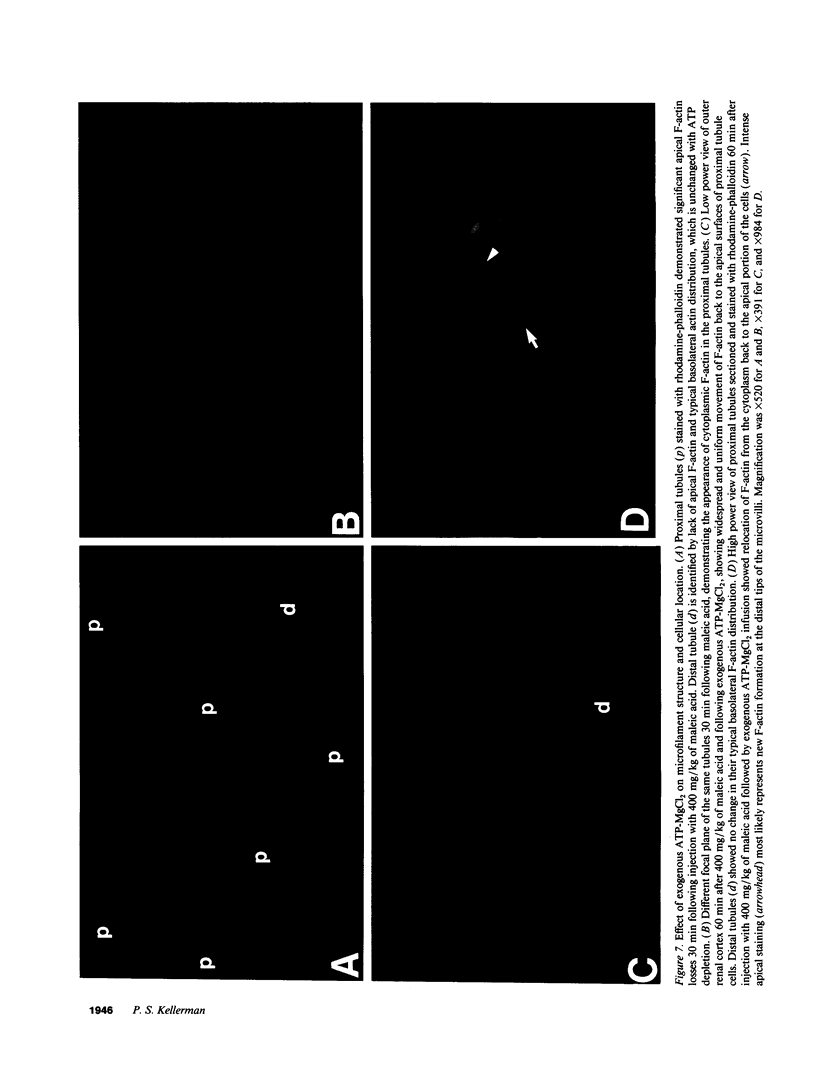
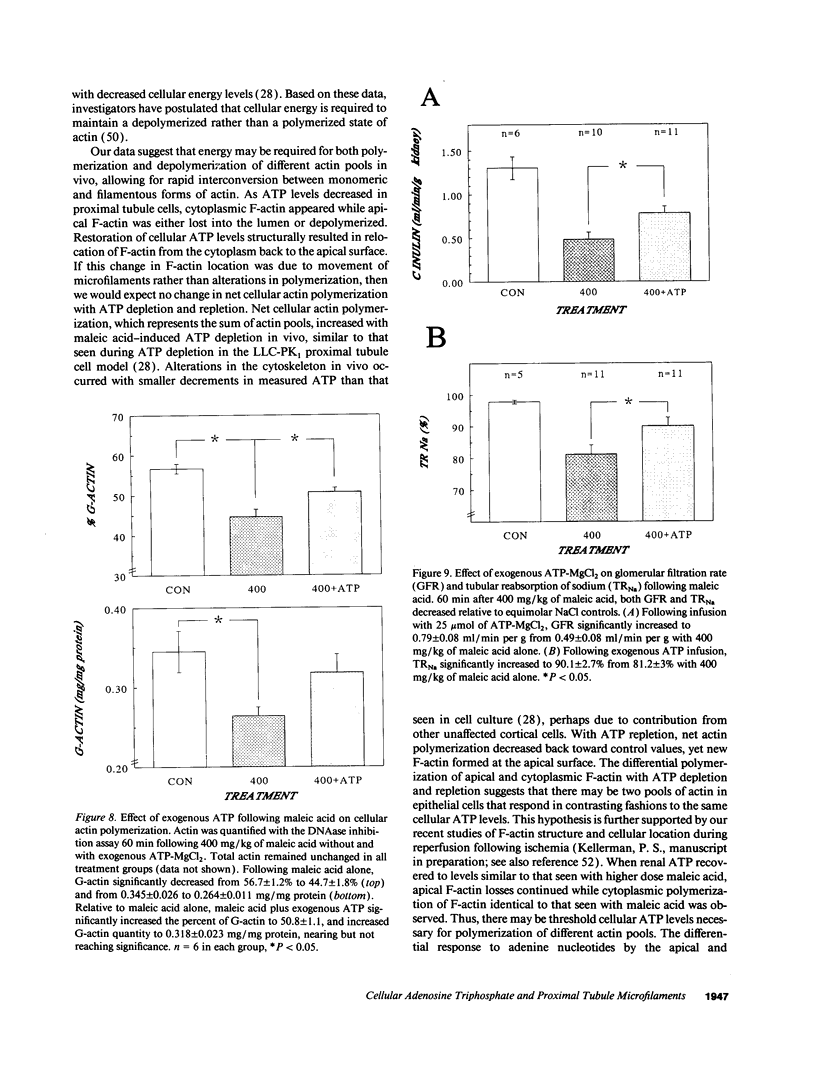
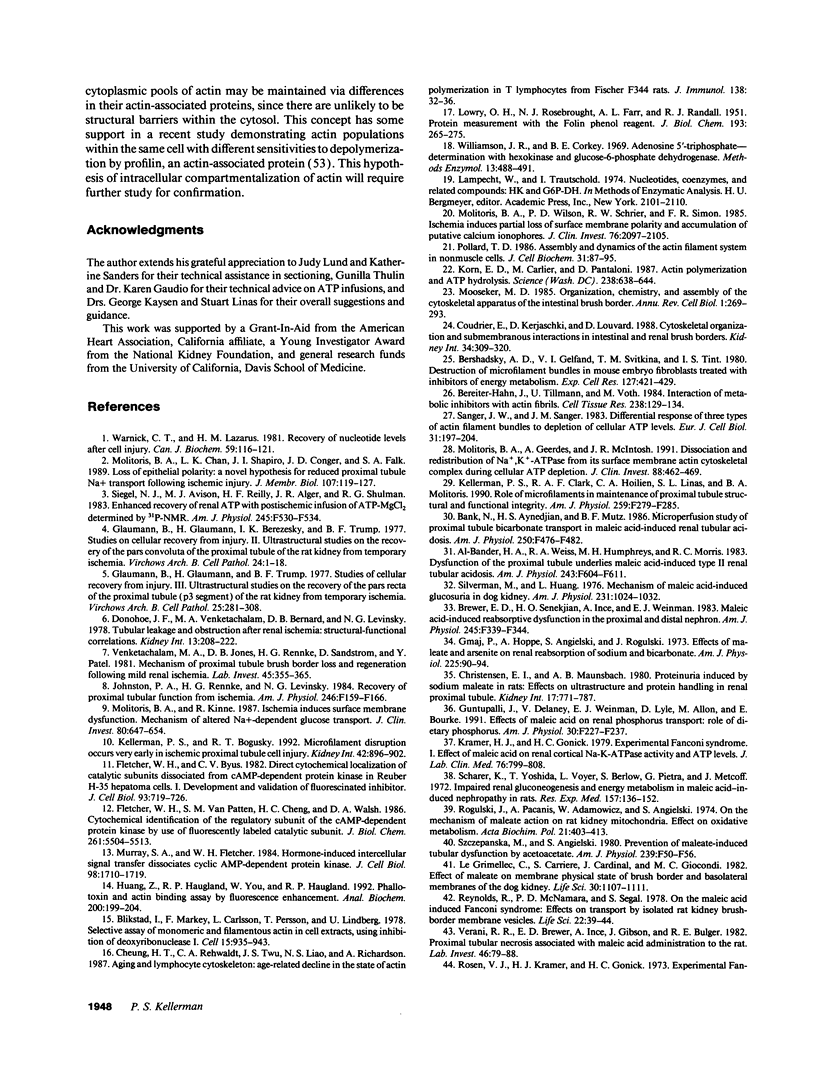
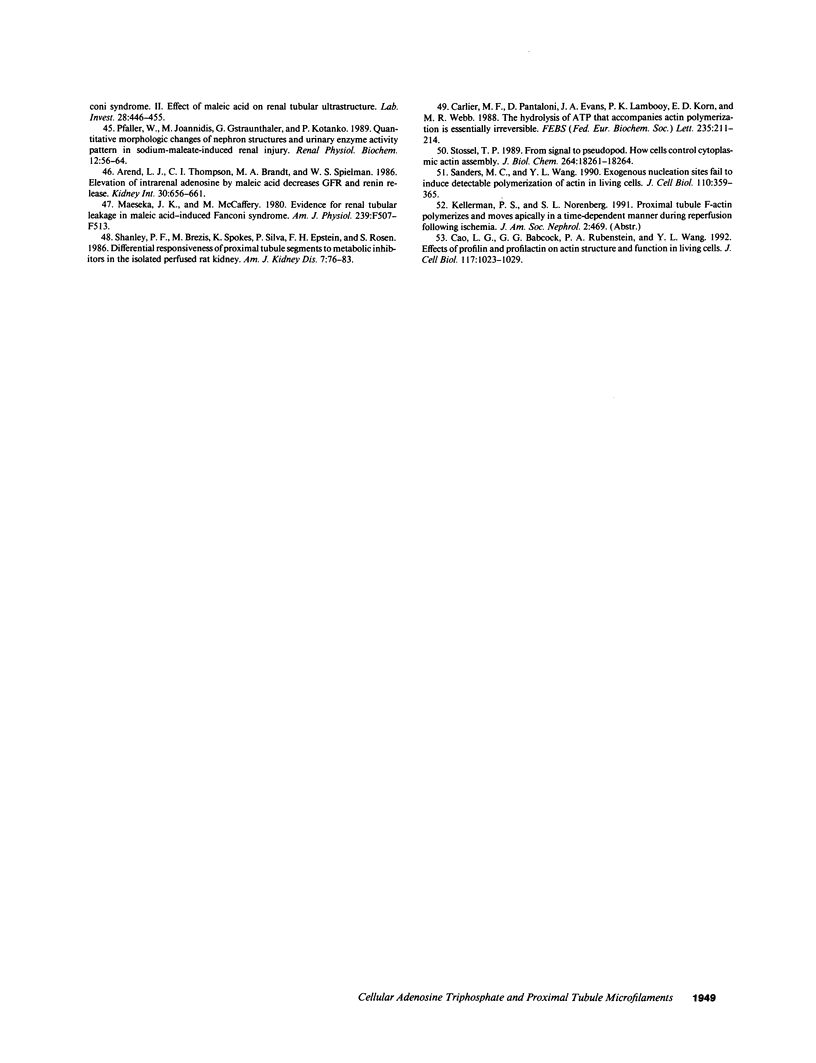
Images in this article
Selected References
These references are in PubMed. This may not be the complete list of references from this article.
- Al-Bander H. A., Weiss R. A., Humphreys M. H., Morris R. C., Jr Dysfunction of the proximal tubule underlies maleic acid-induced type II renal tubular acidosis. Am J Physiol. 1982 Dec;243(6):F604–F611. doi: 10.1152/ajprenal.1982.243.6.F604. [DOI] [PubMed] [Google Scholar]
- Arend L. J., Thompson C. I., Brandt M. A., Spielman W. S. Elevation of intrarenal adenosine by maleic acid decreases GFR and renin release. Kidney Int. 1986 Nov;30(5):656–661. doi: 10.1038/ki.1986.236. [DOI] [PubMed] [Google Scholar]
- Bank N., Aynedjian H. S., Mutz B. F. Microperfusion study of proximal tubule bicarbonate transport in maleic acid-induced renal tubular acidosis. Am J Physiol. 1986 Mar;250(3 Pt 2):F476–F482. doi: 10.1152/ajprenal.1986.250.3.F476. [DOI] [PubMed] [Google Scholar]
- Bereiter-Hahn J., Tillmann U., Vöth M. Interaction of metabolic inhibitors with actin fibrils. Cell Tissue Res. 1984;238(1):129–134. doi: 10.1007/BF00215153. [DOI] [PubMed] [Google Scholar]
- Bershadsky A. D., Gelfand V. I., Svitkina T. M., Tint I. S. Destruction of microfilament bundles in mouse embryo fibroblasts treated with inhibitors of energy metabolism. Exp Cell Res. 1980 Jun;127(2):421–429. doi: 10.1016/0014-4827(80)90446-2. [DOI] [PubMed] [Google Scholar]
- Blikstad I., Markey F., Carlsson L., Persson T., Lindberg U. Selective assay of monomeric and filamentous actin in cell extracts, using inhibition of deoxyribonuclease I. Cell. 1978 Nov;15(3):935–943. doi: 10.1016/0092-8674(78)90277-5. [DOI] [PubMed] [Google Scholar]
- Brewer E. D., Senekjian H. O., Ince A., Weinman E. J. Maleic acid-induced reabsorptive dysfunction in the proximal and distal nephron. Am J Physiol. 1983 Sep;245(3):F339–F344. doi: 10.1152/ajprenal.1983.245.3.F339. [DOI] [PubMed] [Google Scholar]
- Cao L. G., Babcock G. G., Rubenstein P. A., Wang Y. L. Effects of profilin and profilactin on actin structure and function in living cells. J Cell Biol. 1992 Jun;117(5):1023–1029. doi: 10.1083/jcb.117.5.1023. [DOI] [PMC free article] [PubMed] [Google Scholar]
- Carlier M. F., Pantaloni D., Evans J. A., Lambooy P. K., Korn E. D., Webb M. R. The hydrolysis of ATP that accompanies actin polymerization is essentially irreversible. FEBS Lett. 1988 Aug 1;235(1-2):211–214. doi: 10.1016/0014-5793(88)81264-x. [DOI] [PubMed] [Google Scholar]
- Cheung H. T., Rehwaldt C. A., Twu J. S., Liao N. S., Richardson A. Aging and lymphocyte cytoskeleton: age-related decline in the state of actin polymerization in T lymphocytes from Fischer F344 rats. J Immunol. 1987 Jan 1;138(1):32–36. [PubMed] [Google Scholar]
- Christensen E. I., Maunsbach A. B. Proteinuria induced by sodium maleate in rats: effects on ultrastructure and protein handling in renal proximal tubule. Kidney Int. 1980 Jun;17(6):771–787. doi: 10.1038/ki.1980.90. [DOI] [PubMed] [Google Scholar]
- Coudrier E., Kerjaschki D., Louvard D. Cytoskeleton organization and submembranous interactions in intestinal and renal brush borders. Kidney Int. 1988 Sep;34(3):309–320. doi: 10.1038/ki.1988.183. [DOI] [PubMed] [Google Scholar]
- Donohoe J. F., Venkatachalam M. A., Bernard D. B., Levinsky N. G. Tubular leakage and obstruction after renal ischemia: structural-functional correlations. Kidney Int. 1978 Mar;13(3):208–222. doi: 10.1038/ki.1978.31. [DOI] [PubMed] [Google Scholar]
- Fletcher W. H., Byus C. V. Direct cytochemical localization of catalytic subunits dissociated from cAMP-dependent protein kinase in Reuber H-35 hepatoma cells. I. Development and validation of fluorescinated inhibitor. J Cell Biol. 1982 Jun;93(3):719–726. doi: 10.1083/jcb.93.3.719. [DOI] [PMC free article] [PubMed] [Google Scholar]
- Fletcher W. H., Van Patten S. M., Cheng H. C., Walsh D. A. Cytochemical identification of the regulatory subunit of the cAMP-dependent protein kinase by use of fluorescently labeled catalytic subunit. Examination of protein kinase dissociation in hepatoma cells responding to 8-Br-cAMP stimulation. J Biol Chem. 1986 Apr 25;261(12):5504–5513. [PubMed] [Google Scholar]
- Glaumann B., Glaumann H., Berezesky I. K., Trump B. F. Studies on cellular recovery from injury. II. Ultrastructural studies on the recovery of the pars convoluta of the proximal tubule of the rate kidney from temporary ischemia. Virchows Arch B Cell Pathol. 1977 May 3;24(1):1–18. [PubMed] [Google Scholar]
- Glaumann B., Glaumann H., Trump B. F. Studies of cellular recovery from injury. III. Ultrastructural studies on the recovery of the pars recta of the proximal tubule (P3 segment) of the rat kidney from temporary ischemia. Virchows Arch B Cell Pathol. 1977 Nov 21;25(4):281–308. [PubMed] [Google Scholar]
- Gmaj P., Hoppe A., Angielski S., Rogulski J. Effects of maleate and arsenite on renal reabsorption of sodium and bicarbonate. Am J Physiol. 1973 Jul;225(1):90–94. doi: 10.1152/ajplegacy.1973.225.1.90. [DOI] [PubMed] [Google Scholar]
- Guntupalli J., Delaney V., Weinman E. J., Lyle D., Allon M., Bourke E. Effects of maleic acid on renal phosphorus transport: role of dietary phosphorus. Am J Physiol. 1991 Aug;261(2 Pt 2):F227–F237. doi: 10.1152/ajprenal.1991.261.2.F227. [DOI] [PubMed] [Google Scholar]
- Huang Z. J., Haugland R. P., You W. M., Haugland R. P. Phallotoxin and actin binding assay by fluorescence enhancement. Anal Biochem. 1992 Jan;200(1):199–204. doi: 10.1016/0003-2697(92)90299-m. [DOI] [PubMed] [Google Scholar]
- Johnston P. A., Rennke H., Levinsky N. G. Recovery of proximal tubular function from ischemic injury. Am J Physiol. 1984 Feb;246(2 Pt 2):F159–F166. doi: 10.1152/ajprenal.1984.246.2.F159. [DOI] [PubMed] [Google Scholar]
- Kellerman P. S., Bogusky R. T. Microfilament disruption occurs very early in ischemic proximal tubule cell injury. Kidney Int. 1992 Oct;42(4):896–902. doi: 10.1038/ki.1992.366. [DOI] [PubMed] [Google Scholar]
- Kellerman P. S., Clark R. A., Hoilien C. A., Linas S. L., Molitoris B. A. Role of microfilaments in maintenance of proximal tubule structural and functional integrity. Am J Physiol. 1990 Aug;259(2 Pt 2):F279–F285. doi: 10.1152/ajprenal.1990.259.2.F279. [DOI] [PubMed] [Google Scholar]
- Korn E. D., Carlier M. F., Pantaloni D. Actin polymerization and ATP hydrolysis. Science. 1987 Oct 30;238(4827):638–644. doi: 10.1126/science.3672117. [DOI] [PubMed] [Google Scholar]
- Kramer H. J., Gonick H. C. Experimental Fanconi syndrome. I. Effect of maleic acid on renal cortical Na-K-ATPase activity and ATP levels. J Lab Clin Med. 1970 Nov;76(5):799–808. [PubMed] [Google Scholar]
- LOWRY O. H., ROSEBROUGH N. J., FARR A. L., RANDALL R. J. Protein measurement with the Folin phenol reagent. J Biol Chem. 1951 Nov;193(1):265–275. [PubMed] [Google Scholar]
- Le Grimellec C., Carrière S., Cardinal J., Giocondi M. C. Effect of maleate on membrane physical state of brush border and basolateral membranes of the dog kidney. Life Sci. 1982 Mar 29;30(13):1107–1111. doi: 10.1016/0024-3205(82)90531-8. [DOI] [PubMed] [Google Scholar]
- Maesaka J. K., McCaffery M. Evidence for renal tubular leakage in maleic acid-induced Fanconi syndrome. Am J Physiol. 1980 Nov;239(5):F507–F513. doi: 10.1152/ajprenal.1980.239.5.F507. [DOI] [PubMed] [Google Scholar]
- Molitoris B. A., Chan L. K., Shapiro J. I., Conger J. D., Falk S. A. Loss of epithelial polarity: a novel hypothesis for reduced proximal tubule Na+ transport following ischemic injury. J Membr Biol. 1989 Feb;107(2):119–127. doi: 10.1007/BF01871717. [DOI] [PubMed] [Google Scholar]
- Molitoris B. A., Geerdes A., McIntosh J. R. Dissociation and redistribution of Na+,K(+)-ATPase from its surface membrane actin cytoskeletal complex during cellular ATP depletion. J Clin Invest. 1991 Aug;88(2):462–469. doi: 10.1172/JCI115326. [DOI] [PMC free article] [PubMed] [Google Scholar]
- Molitoris B. A., Kinne R. Ischemia induces surface membrane dysfunction. Mechanism of altered Na+-dependent glucose transport. J Clin Invest. 1987 Sep;80(3):647–654. doi: 10.1172/JCI113117. [DOI] [PMC free article] [PubMed] [Google Scholar]
- Molitoris B. A., Wilson P. D., Schrier R. W., Simon F. R. Ischemia induces partial loss of surface membrane polarity and accumulation of putative calcium ionophores. J Clin Invest. 1985 Dec;76(6):2097–2105. doi: 10.1172/JCI112214. [DOI] [PMC free article] [PubMed] [Google Scholar]
- Murray S. A., Fletcher W. H. Hormone-induced intercellular signal transfer dissociates cyclic AMP-dependent protein kinase. J Cell Biol. 1984 May;98(5):1710–1719. doi: 10.1083/jcb.98.5.1710. [DOI] [PMC free article] [PubMed] [Google Scholar]
- Pfaller W., Joannidis M., Gstraunthaler G., Kotanko P. Quantitative morphologic changes in nephron structures and urinary enzyme activity pattern in sodium-maleate-induced renal injury. Ren Physiol Biochem. 1989 Jan-Feb;12(1):56–64. doi: 10.1159/000173180. [DOI] [PubMed] [Google Scholar]
- Pollard T. D. Assembly and dynamics of the actin filament system in nonmuscle cells. J Cell Biochem. 1986;31(2):87–95. doi: 10.1002/jcb.240310202. [DOI] [PubMed] [Google Scholar]
- Reynolds R., McNamara P. D., Segal S. On the maleic acid induced Fanconi syndrome: effects on transport by isolated rat kidney brushborder membrane vesicles. Life Sci. 1978 Jan;22(1):39–43. doi: 10.1016/0024-3205(78)90409-5. [DOI] [PubMed] [Google Scholar]
- Rigulski J., Pacanis A., Adamowicz W., Angielski S. On the mechanism of maleate action on rat kidney mitochondria. Effect on oxidative metabolism. Acta Biochim Pol. 1974;21(4):403–413. [PubMed] [Google Scholar]
- Sanders M. C., Wang Y. L. Exogenous nucleation sites fail to induce detectable polymerization of actin in living cells. J Cell Biol. 1990 Feb;110(2):359–365. doi: 10.1083/jcb.110.2.359. [DOI] [PMC free article] [PubMed] [Google Scholar]
- Sanger J. W., Sanger J. M., Jockusch B. M. Differential response of three types of actin filament bundles to depletion of cellular ATP levels. Eur J Cell Biol. 1983 Sep;31(2):197–204. [PubMed] [Google Scholar]
- Schärer K., Yoshida T., Voyer L., Berlow S., Pietra G., Metcoff J. Impaired renal gluconeogenesis and energy metabolism in maleic acid-induced nephropathy in rats. Res Exp Med (Berl) 1972;157(2):136–152. doi: 10.1007/BF01851694. [DOI] [PubMed] [Google Scholar]
- Shanley P. F., Brezis M., Spokes K., Silva P., Epstein F. H., Rosen S. Differential responsiveness of proximal tubule segments to metabolic inhibitors in the isolated perfused rat kidney. Am J Kidney Dis. 1986 Jan;7(1):76–83. doi: 10.1016/s0272-6386(86)80059-2. [DOI] [PubMed] [Google Scholar]
- Siegel N. J., Avison M. J., Reilly H. F., Alger J. R., Shulman R. G. Enhanced recovery of renal ATP with postischemic infusion of ATP-MgCl2 determined by 31P-NMR. Am J Physiol. 1983 Oct;245(4):F530–F534. doi: 10.1152/ajprenal.1983.245.4.F530. [DOI] [PubMed] [Google Scholar]
- Silverman M., Huang L. Mechanism of maleic acid-induced glucosuria in dog kidney. Am J Physiol. 1976 Oct;231(4):1024–1032. doi: 10.1152/ajplegacy.1976.231.4.1024. [DOI] [PubMed] [Google Scholar]
- Stossel T. P. From signal to pseudopod. How cells control cytoplasmic actin assembly. J Biol Chem. 1989 Nov 5;264(31):18261–18264. [PubMed] [Google Scholar]
- Szczepańska M., Angielski S. Prevention of maleate-induced tubular dysfunction by acetoacetate. Am J Physiol. 1980 Jul;239(1):F50–F56. doi: 10.1152/ajprenal.1980.239.1.F50. [DOI] [PubMed] [Google Scholar]
- Venkatachalam M. A., Jones D. B., Rennke H. G., Sandstrom D., Patel Y. Mechanism of proximal tubule brush border loss and regeneration following mild renal ischemia. Lab Invest. 1981 Oct;45(4):355–365. [PubMed] [Google Scholar]
- Verani R. R., Brewer E. D., Ince A., Gibson J., Bulger R. E. Proximal tubular necrosis associated with maleic acid administration to the rat. Lab Invest. 1982 Jan;46(1):79–88. [PubMed] [Google Scholar]
- Warnick C. T., Lazarus H. M. Recovery of nucleotide levels after cell injury. Can J Biochem. 1981 Feb;59(2):116–121. doi: 10.1139/o81-017. [DOI] [PubMed] [Google Scholar]