Abstract
The cells of the marine bacterium Ant-300 were found to take up arginine when this substrate was at low concentrations. The cells possessed an uptake system(s) that specifically transported l-arginine. The kinetic parameters for uptake appeared to differ when the cells were exposed to nanomolar and micromolar concentrations of the amino acid. Uptake over this concentration range functioned in the absence of an exogenous energy source, even after the cells had been preincubated in unsupplemented artificial seawater. Respiratory activity appeared to be a more important driving force for arginine uptake than adenosine 5′-triphosphate hydrolysis. The cells also exhibited chemotaxis toward l-arginine. The minimum arginine concentration needed to elicit a chemotactic response was between 10−5 and 10−6 M. It is proposed that the capture of arginine by cells of Ant-300 in nutrient-depleted waters, which are typical of the open ocean, proceeds via high-affinity active transport, whereas in substrate-enriched seawater, capture involves chemotaxis and an active transport mechanism with reduced affinity for the substrate.
Full text
PDF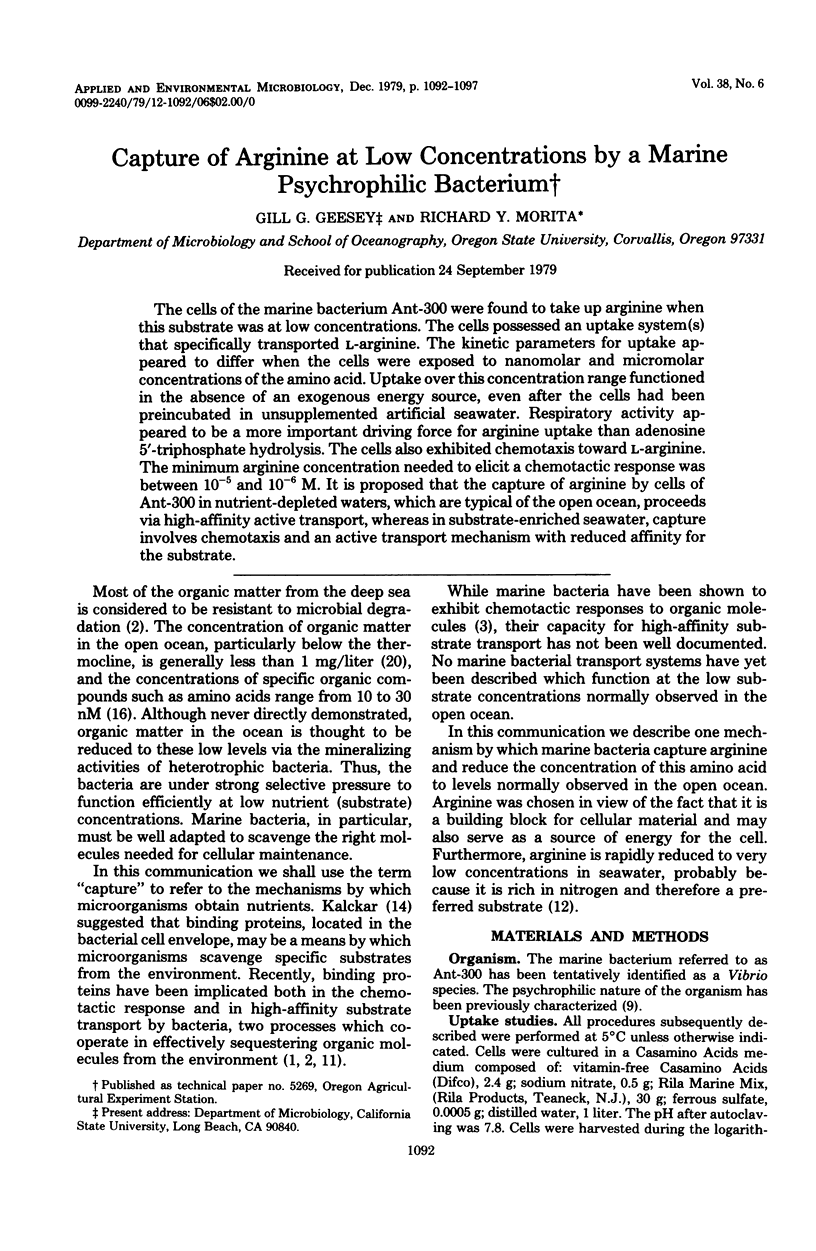
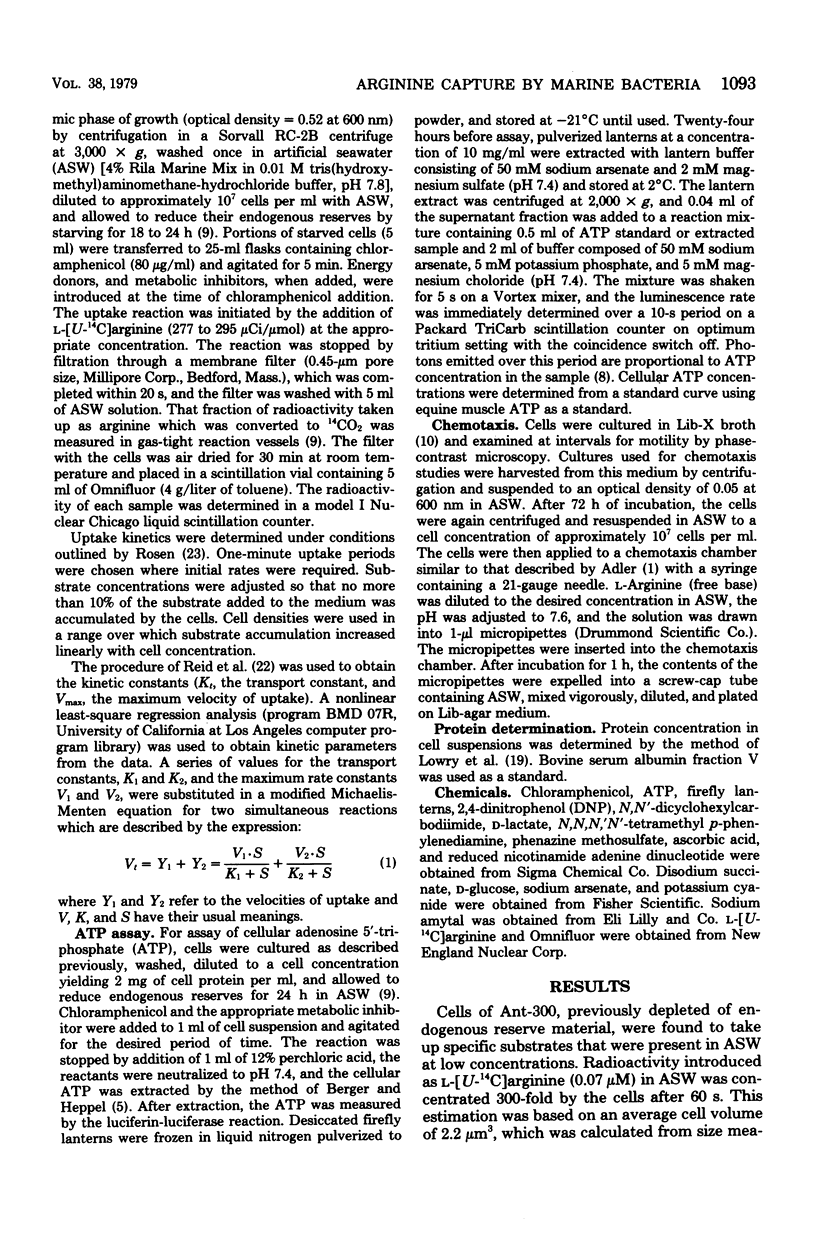
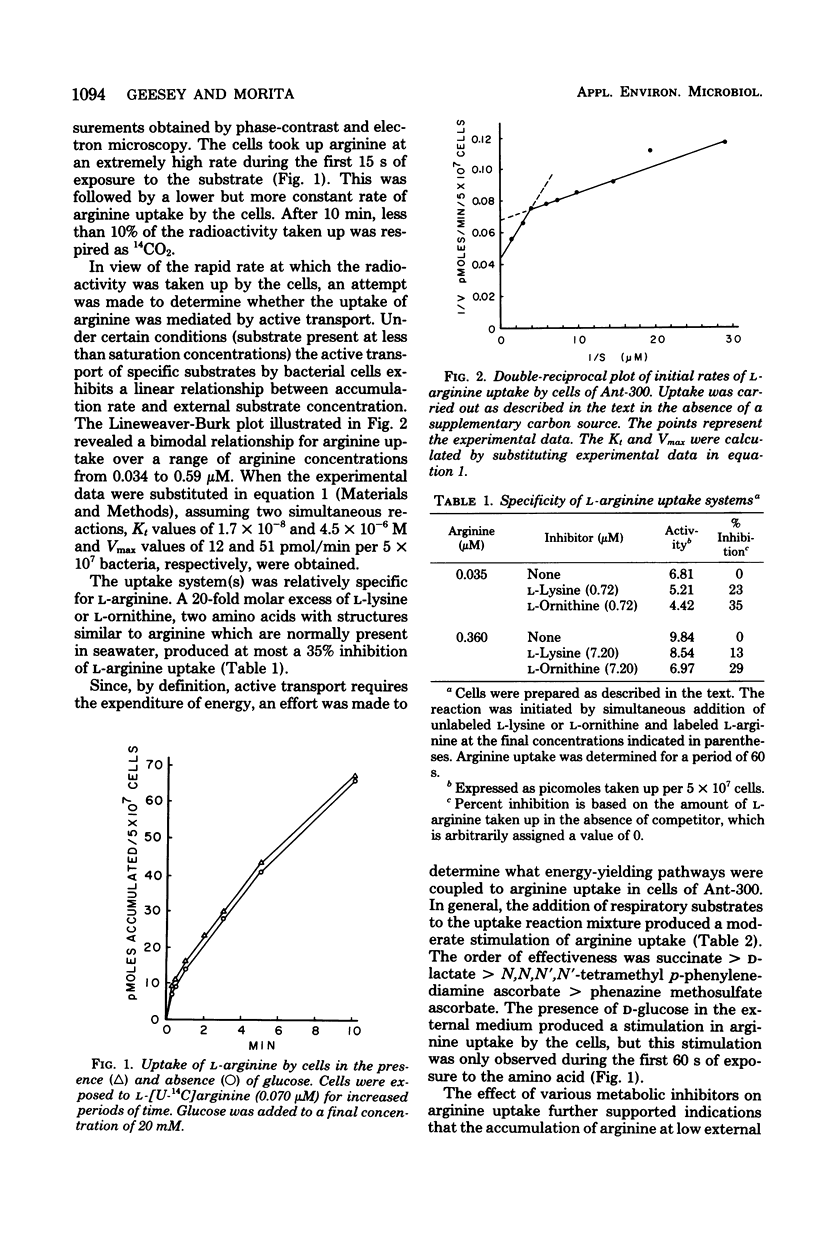
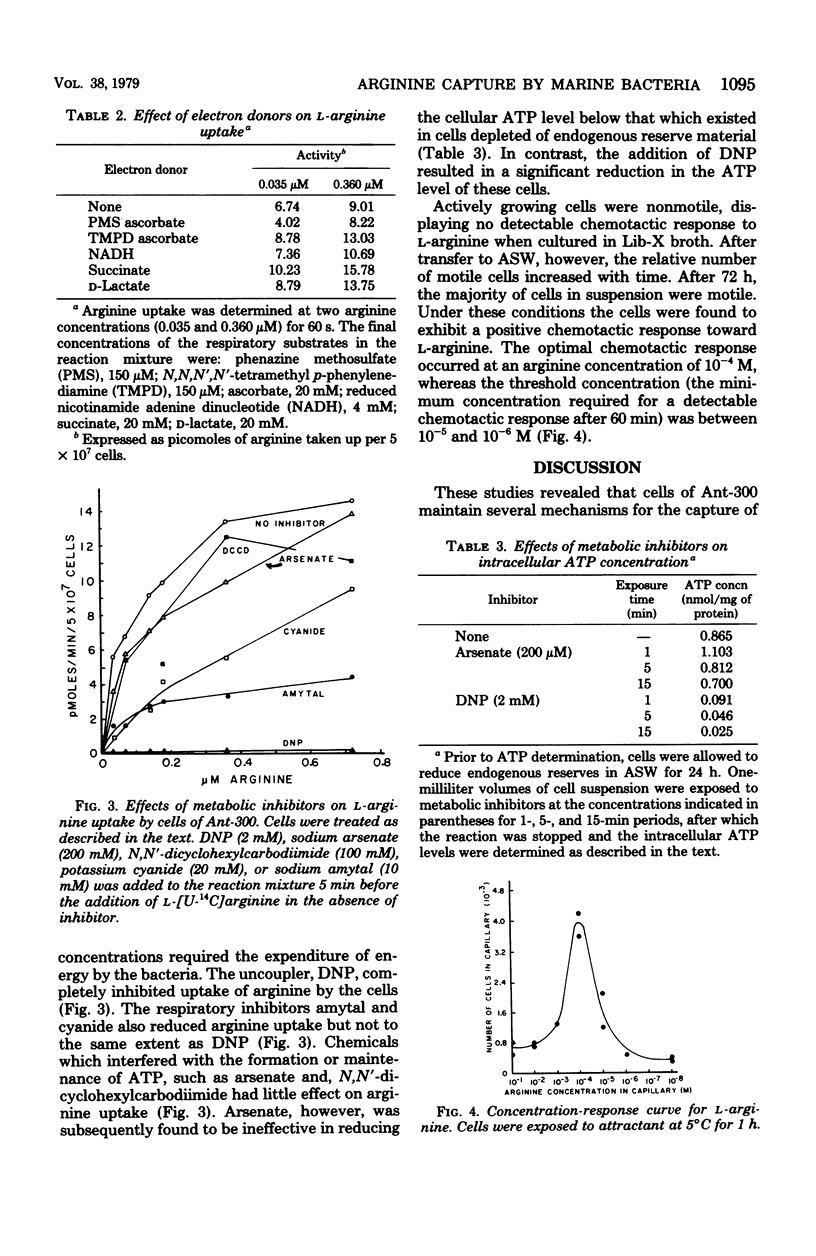
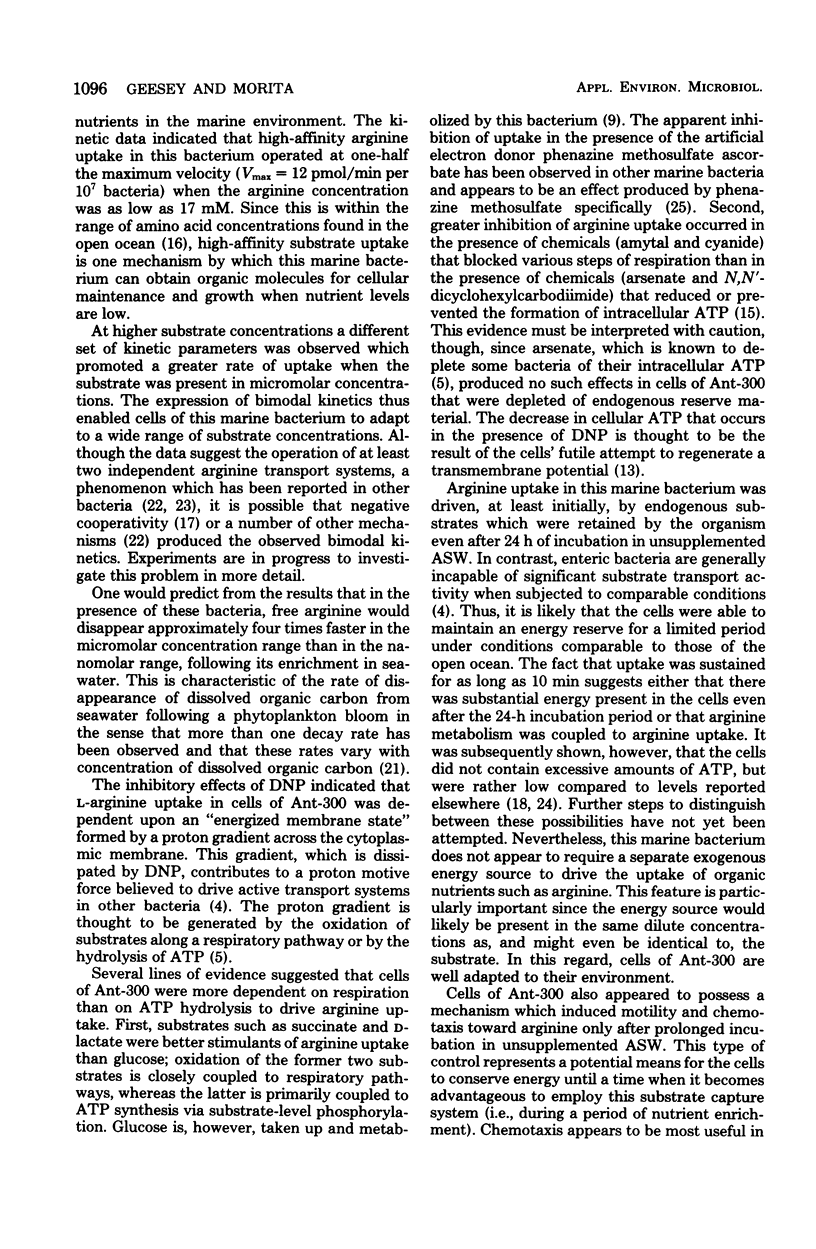
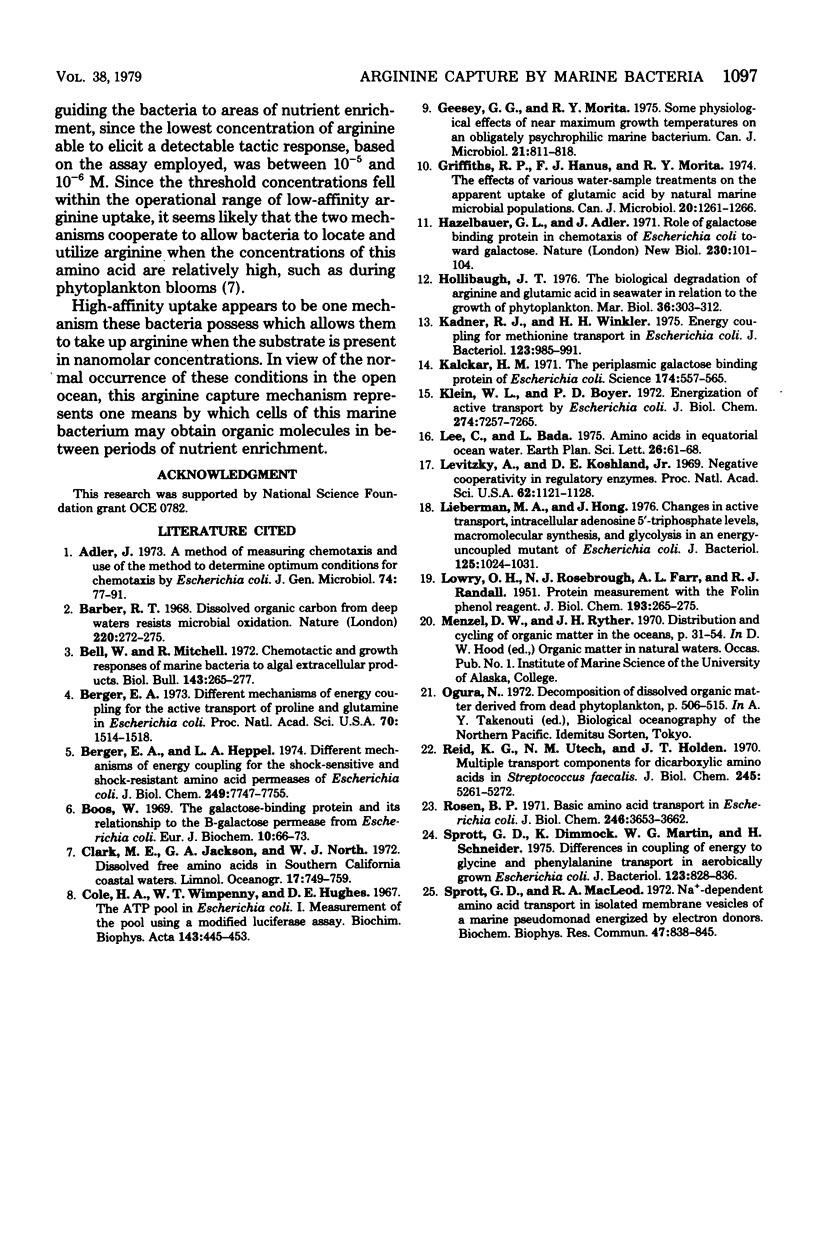
Selected References
These references are in PubMed. This may not be the complete list of references from this article.
- Adler J. A method for measuring chemotaxis and use of the method to determine optimum conditions for chemotaxis by Escherichia coli. J Gen Microbiol. 1973 Jan;74(1):77–91. doi: 10.1099/00221287-74-1-77. [DOI] [PubMed] [Google Scholar]
- Barber R. T. Dissolved organic carbon from deep waters resists microbial oxidation. Nature. 1968 Oct 19;220(5164):274–275. doi: 10.1038/220274a0. [DOI] [PubMed] [Google Scholar]
- Berger E. A. Different mechanisms of energy coupling for the active transport of proline and glutamine in Escherichia coli. Proc Natl Acad Sci U S A. 1973 May;70(5):1514–1518. doi: 10.1073/pnas.70.5.1514. [DOI] [PMC free article] [PubMed] [Google Scholar]
- Berger E. A., Heppel L. A. Different mechanisms of energy coupling for the shock-sensitive and shock-resistant amino acid permeases of Escherichia coli. J Biol Chem. 1974 Dec 25;249(24):7747–7755. [PubMed] [Google Scholar]
- Boos W. The galactose binding protein and its relationship to the beta-methylgalactoside permease from Escherichia coli. Eur J Biochem. 1969 Aug;10(1):66–73. doi: 10.1111/j.1432-1033.1969.tb00656.x. [DOI] [PubMed] [Google Scholar]
- Cole H. A., Wimpenny J. W., Hughes D. E. The ATP pool in Escherichia coli. I. Measurement of the pool using modified luciferase assay. Biochim Biophys Acta. 1967;143(3):445–453. doi: 10.1016/0005-2728(67)90050-3. [DOI] [PubMed] [Google Scholar]
- Geesey G. G., Morita R. Y. Some physiological effects of near-maximum growth temperatures on an obligately psychrophilic marine bacterium. Can J Microbiol. 1975 Jun;21(6):811–818. doi: 10.1139/m75-120. [DOI] [PubMed] [Google Scholar]
- Griffiths R. P., Hanus F. J., Morita R. Y. The effects of various water-sample treatments on the apparent uptake of glutamic acid by natural marine microbial populations. Can J Microbiol. 1974 Sep;20(9):1261–1266. doi: 10.1139/m74-194. [DOI] [PubMed] [Google Scholar]
- Hazelbauer G. L., Adler J. Role of the galactose binding protein in chemotaxis of Escherichia coli toward galactose. Nat New Biol. 1971 Mar 24;230(12):101–104. doi: 10.1038/newbio230101a0. [DOI] [PubMed] [Google Scholar]
- Kadner R. J., Winkler H. H. Energy coupling for methionine transport in Escherichia coli. J Bacteriol. 1975 Sep;123(3):985–991. doi: 10.1128/jb.123.3.985-991.1975. [DOI] [PMC free article] [PubMed] [Google Scholar]
- Kalckar H. M. The periplasmic galactose binding protein of Escherichia coli. Science. 1971 Nov 5;174(4009):557–565. doi: 10.1126/science.174.4009.557. [DOI] [PubMed] [Google Scholar]
- Klein W. L., Boyer P. D. Energization of active transport by Escherichia coli. J Biol Chem. 1972 Nov 25;247(22):7257–7265. [PubMed] [Google Scholar]
- LOWRY O. H., ROSEBROUGH N. J., FARR A. L., RANDALL R. J. Protein measurement with the Folin phenol reagent. J Biol Chem. 1951 Nov;193(1):265–275. [PubMed] [Google Scholar]
- Levitzki A., Koshland D. E., Jr Negative cooperativity in regulatory enzymes. Proc Natl Acad Sci U S A. 1969 Apr;62(4):1121–1128. doi: 10.1073/pnas.62.4.1121. [DOI] [PMC free article] [PubMed] [Google Scholar]
- Lieberman M. A., Hong J. S. Changes in active transport, intracellular adenosine 5'-triphosphate levels, macromolecular syntheses, and glycolysis in an energy-uncoupled mutant of Escherichia coli. J Bacteriol. 1976 Mar;125(3):1024–1031. doi: 10.1128/jb.125.3.1024-1031.1976. [DOI] [PMC free article] [PubMed] [Google Scholar]
- Reid K. G., Utech N. M., Holden J. T. Multiple transport components for dicarboxylic amino acids in Streptococcus faecalis. J Biol Chem. 1970 Oct 25;245(20):5261–5272. [PubMed] [Google Scholar]
- Rosen B. P. Basic amino acid transport in Escherichia coli. J Biol Chem. 1971 Jun 10;246(11):3653–3662. [PubMed] [Google Scholar]
- Sprott G. D., Dimock K., Martin W. G., Schneider H. Differences in coupling of energy to glycine and phenylalanine transport in aerobically grown Escherichia coli. J Bacteriol. 1975 Sep;123(3):828–836. doi: 10.1128/jb.123.3.828-836.1975. [DOI] [PMC free article] [PubMed] [Google Scholar]
- Sprott G. D., MacLeod R. A. Na + -dependent amino acid transport in isolated membrane vesicles of a marine pseudomonad energized by electron donors. Biochem Biophys Res Commun. 1972 May 26;47(4):838–845. doi: 10.1016/0006-291x(72)90569-4. [DOI] [PubMed] [Google Scholar]