Abstract
The activity of the Escherichia coli transport proteins for lactose and proline can be altered by changing the redox state of the dithiols in these carriers. A series of lipophilic oxidizing agents has been shown to inhibit and subsequent addition of dithiothreitol to restore full activity. Both systems are irreversibly inhibited by N-ethylmaleimide, but prior addition of oxidizing agents protects against this inhibition. These data, as well as studies on the inhibitory effect of the dithiol-specific reagent phenylarsine oxide, show that the redox-sensitive step is the conversion of a dithiol to a disulfide. Measurement of the initial rate as a function of the lactose and L-proline concentrations shows that the oxidation of a dithiol to a disulfide increases the Km of the carriers for lactose and L-proline. The reduced (dithiol) form of the carrier has a low Km and the oxidized (disulfide) form has a high Km for its substrate. The changes in Km brought about by reduction and oxidation are the same as those that accompany the generation and dissipation, respectively, of an electrochemical proton gradient (delta mu H+). These results support a mechanism in which an delta mu H+ or one of its components alters the ligand affinities of the carrier during a single transport cycle through conversion from the reduced to the oxidized form.
Full text
PDF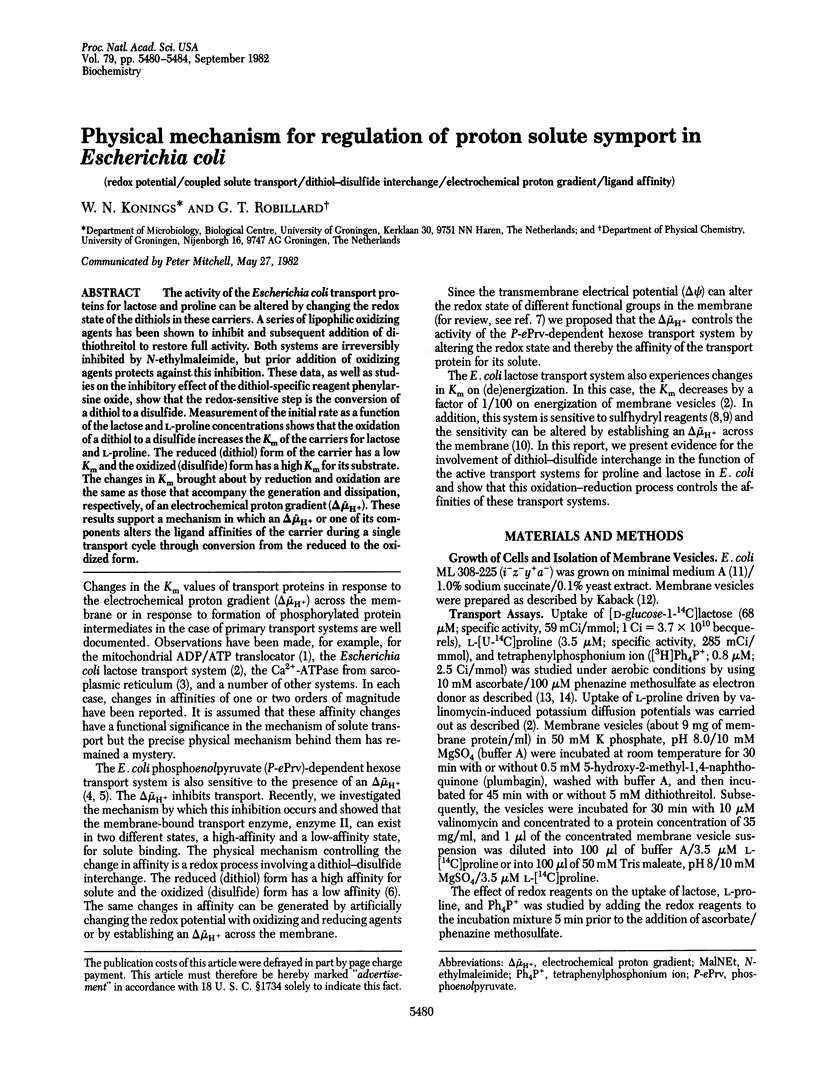
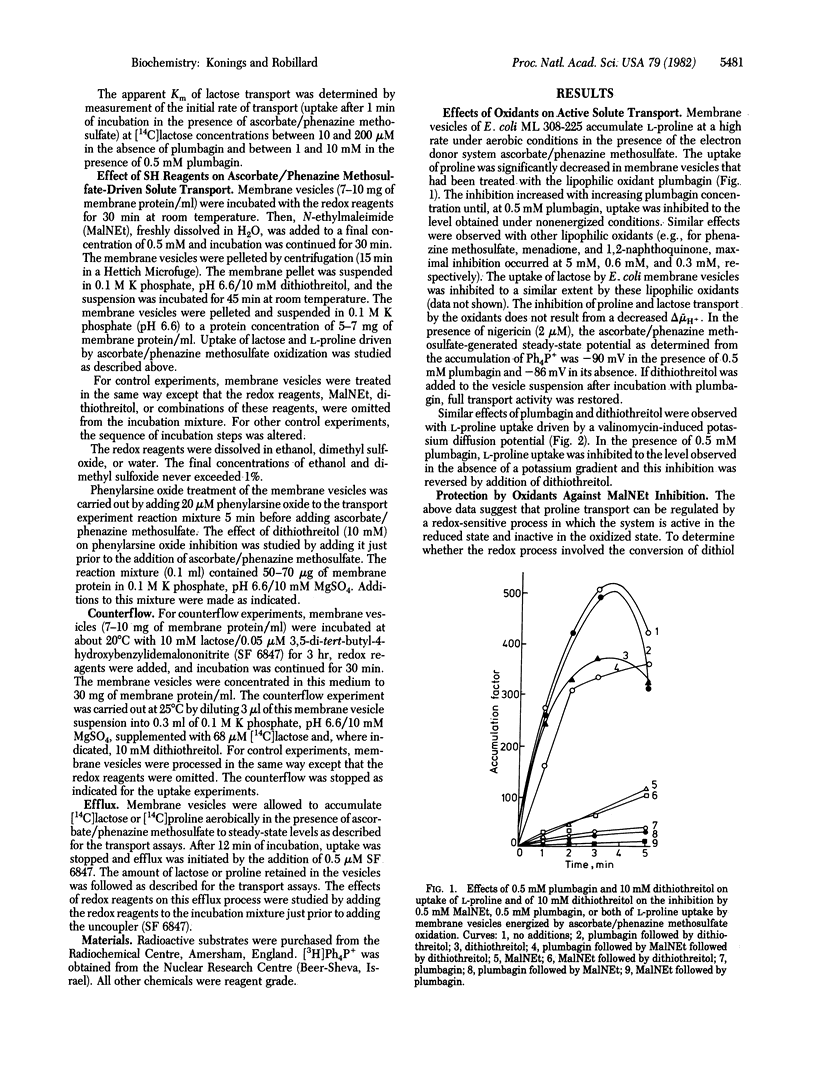
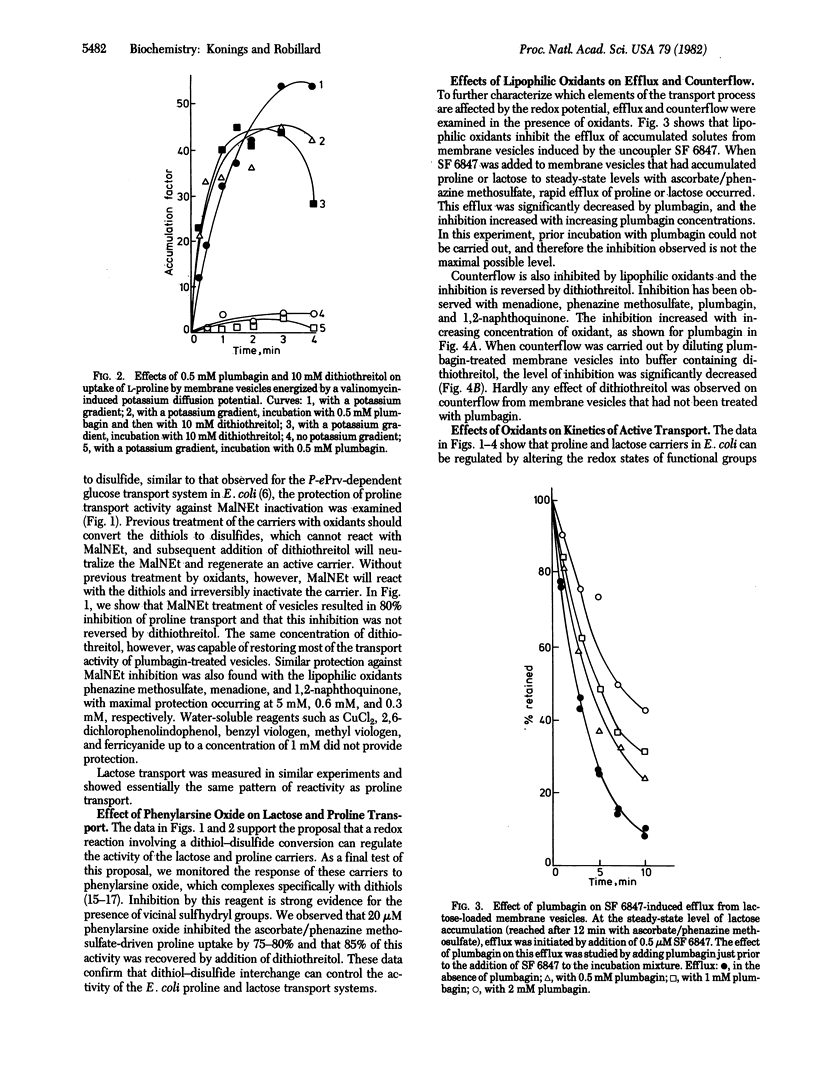
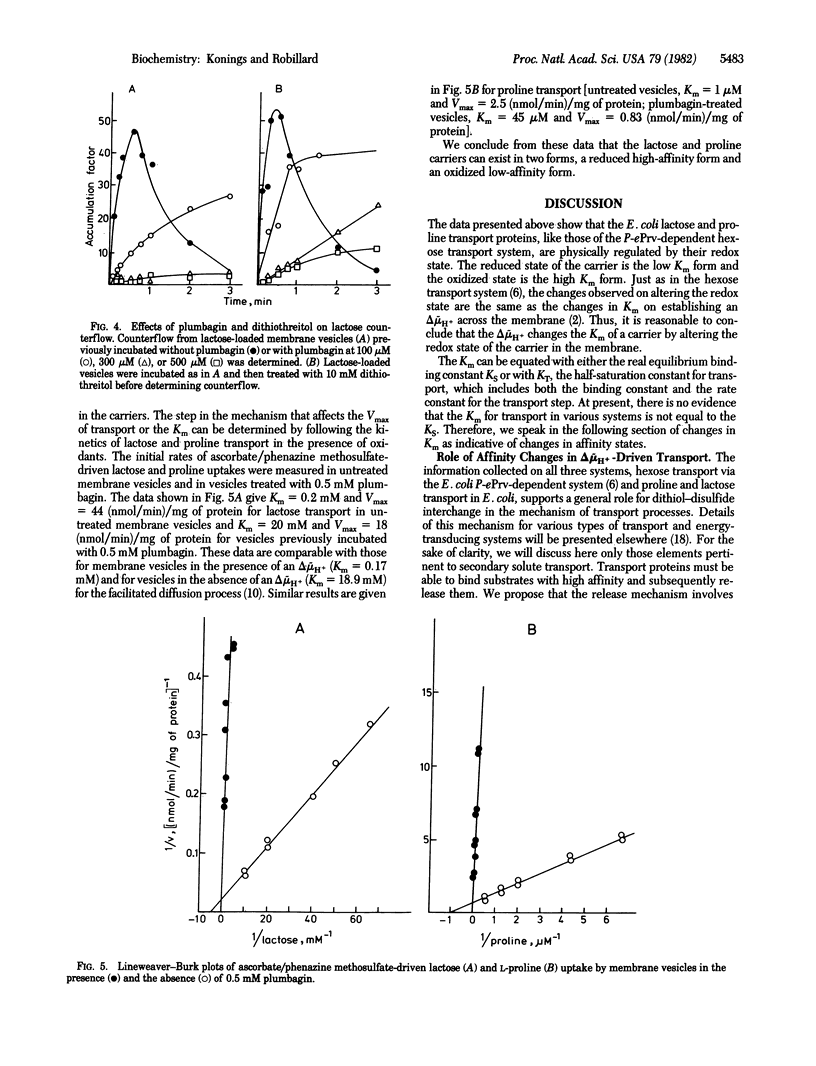
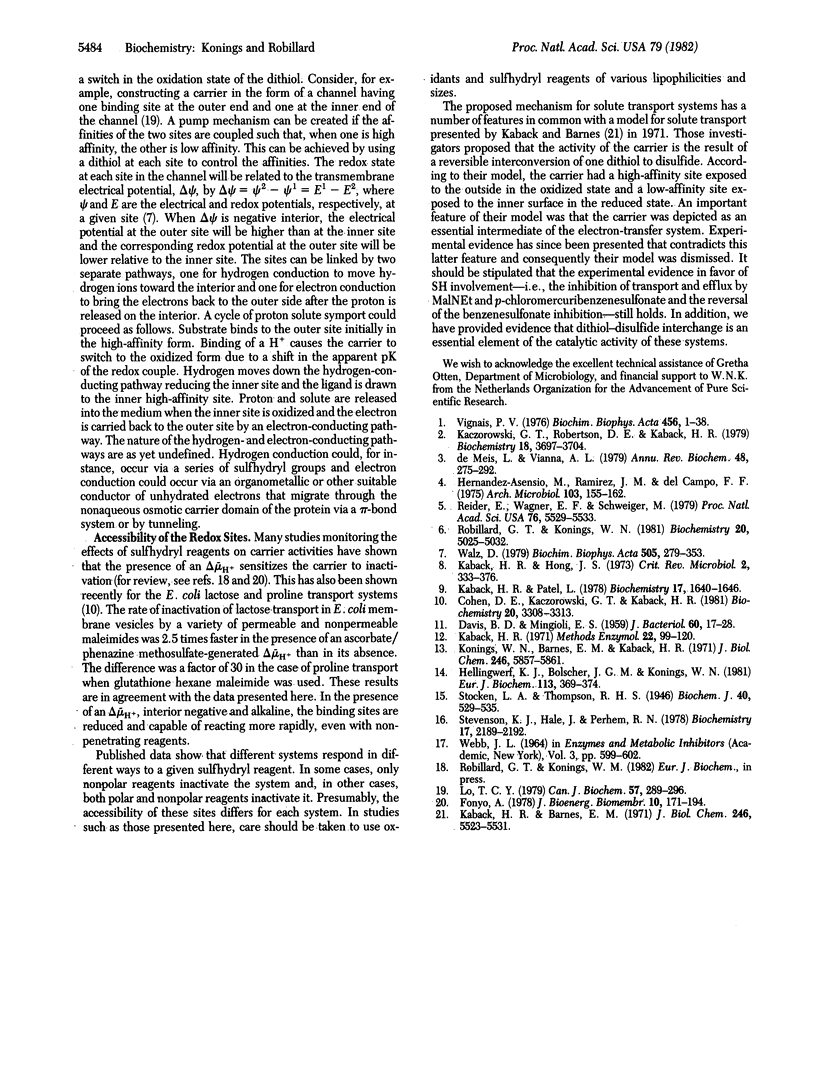
Selected References
These references are in PubMed. This may not be the complete list of references from this article.
- Cohn D. E., Kaczorowski G. J., Kaback H. R. Effect of the proton electrochemical gradient on maleimide inactivation of active transport in Escherichia coli membrane vesicles. Biochemistry. 1981 May 26;20(11):3308–3313. doi: 10.1021/bi00514a050. [DOI] [PubMed] [Google Scholar]
- DAVIS B. D., MINGIOLI E. S. Mutants of Escherichia coli requiring methionine or vitamin B12. J Bacteriol. 1950 Jul;60(1):17–28. doi: 10.1128/jb.60.1.17-28.1950. [DOI] [PMC free article] [PubMed] [Google Scholar]
- Fonyó A. SH-group reagents as tools in the study of mitochondrial anion transport. J Bioenerg Biomembr. 1978 Dec;10(5-6):171–194. doi: 10.1007/BF00743106. [DOI] [PubMed] [Google Scholar]
- Hellingwerf K. J., Bolscher J. G., Konings W. N. The electrochemical proton gradient generated by the fumarate-reductase system in Escherichia coli and its bioenergetic implications. Eur J Biochem. 1981 Jan;113(2):369–374. doi: 10.1111/j.1432-1033.1981.tb05075.x. [DOI] [PubMed] [Google Scholar]
- Hernandez-Asensio M., Ramirez J. M., Del Campo F. F. The control by respiration of the uptake of alpha-methyl glucoside in Escherichia coli K12. Arch Microbiol. 1975 Apr 7;103(2):155–162. doi: 10.1007/BF00436343. [DOI] [PubMed] [Google Scholar]
- Kaback H. R., Barnes E. M., Jr Mechanisms of active transport in isolated membrane vesicles. II. The mechanism of energy coupling between D-lactic dehydrogenase and beta-galactoside transport in membrane preparations from Escherichia coli. J Biol Chem. 1971 Sep 10;246(17):5523–5531. [PubMed] [Google Scholar]
- Kaback H. R., Patel L. The role of functional sulfhydryl groups in active transport in Escherichia coli membrane vesicles. Biochemistry. 1978 May 2;17(9):1640–1646. doi: 10.1021/bi00602a010. [DOI] [PubMed] [Google Scholar]
- Kaczorowski G. J., Robertson D. E., Kaback H. R. Mechanism of lactose translocation in membrane vesicles from Escherichia coli. 2. Effect of imposed delata psi, delta pH, and Delta mu H+. Biochemistry. 1979 Aug 21;18(17):3697–3704. doi: 10.1021/bi00584a010. [DOI] [PubMed] [Google Scholar]
- Konings W. N., Barnes E. M., Jr, Kaback H. R. Mechanisms of active transport in isolated membrane vesicles. 2. The coupling of reduced phenazine methosulfate to the concentrative uptake of beta-galactosides and amino acids. J Biol Chem. 1971 Oct 10;246(19):5857–5861. [PubMed] [Google Scholar]
- Lo T. C. The molecular mechanisms of substrate transport in gram-negative bacteria. Can J Biochem. 1979 Apr;57(4):289–301. doi: 10.1139/o79-037. [DOI] [PubMed] [Google Scholar]
- Reider E., Wagner E. F., Schweiger M. Control of phosphoenolpyruvate-dependent phosphotransferase-mediated sugar transport in Escherichia coli by energization of the cell membrane. Proc Natl Acad Sci U S A. 1979 Nov;76(11):5529–5533. doi: 10.1073/pnas.76.11.5529. [DOI] [PMC free article] [PubMed] [Google Scholar]
- Robillard G. T., Konings W. N. Physical mechanism for regulation of phosphoenolpyruvate-dependent glucose transport activity in Escherichia coli. Biochemistry. 1981 Aug 18;20(17):5025–5032. doi: 10.1021/bi00520a032. [DOI] [PubMed] [Google Scholar]
- Stevenson K. J., Hale G., Perham R. N. Inhibition of pyruvate dehydrogenase multienzyme complex from Escherichia coli with mono- and bifunctional arsenoxides. Biochemistry. 1978 May 30;17(11):2189–2192. doi: 10.1021/bi00604a026. [DOI] [PubMed] [Google Scholar]
- Vignais P. V. Molecular and physiological aspects of adenine nucleotide transport in mitochondria. Biochim Biophys Acta. 1976 Apr 30;456(1):1–38. doi: 10.1016/0304-4173(76)90007-0. [DOI] [PubMed] [Google Scholar]
- Walz D. Thermodynamics of oxidation-reduction reactions and its application to bioenergetics. Biochim Biophys Acta. 1979 Mar 14;505(3-4):279–353. doi: 10.1016/0304-4173(79)90007-7. [DOI] [PubMed] [Google Scholar]
- de Meis L., Vianna A. L. Energy interconversion by the Ca2+-dependent ATPase of the sarcoplasmic reticulum. Annu Rev Biochem. 1979;48:275–292. doi: 10.1146/annurev.bi.48.070179.001423. [DOI] [PubMed] [Google Scholar]