Abstract
Intracellular ATP levels in whole cells of Methanococcus voltae respond to electron transfer coupled to methanogenesis. ATP synthesis can also be induced by an artificially imposed transmembrane electrical potential [formed by electrogenic movement outwards of potassium (induced by valinomycin) or of protons (induced by an uncoupler], or by a pH gradient (acid outside). These results implicate the existence of a reversible ATPase coupled to electrogenic movement of an ion(s) other than potassium or proton, and that ionophores are competent to catalyze ion movement across the cytoplasmic membrane of this organism (which is the sole membrane structure in this species). ATP synthesis driven by electron transfer is, however, insensitive to the addition of such ionophores. These results indicate that although cells possess an ion-translocating ATPase (possibly involved in the maintenance of internal ionic composition homeostasis), methanogenesis-driven ATP synthesis does not involve the intermediacy of a transmembrane ion gradient. Primarily because methane formation has been previously demonstrated to involve true electron transfer, substrate-level phosphorylation (at least in analogy to other systems) has been generally ruled out. The results presented here suggest that at least one methanogenic bacterium may use a direct linkage of ATP synthesis to electron transfer.
Keywords: methanogens, bioenergetics, chemiosmotic theory, substrate-level phosphorylation, electron transport
Full text
PDF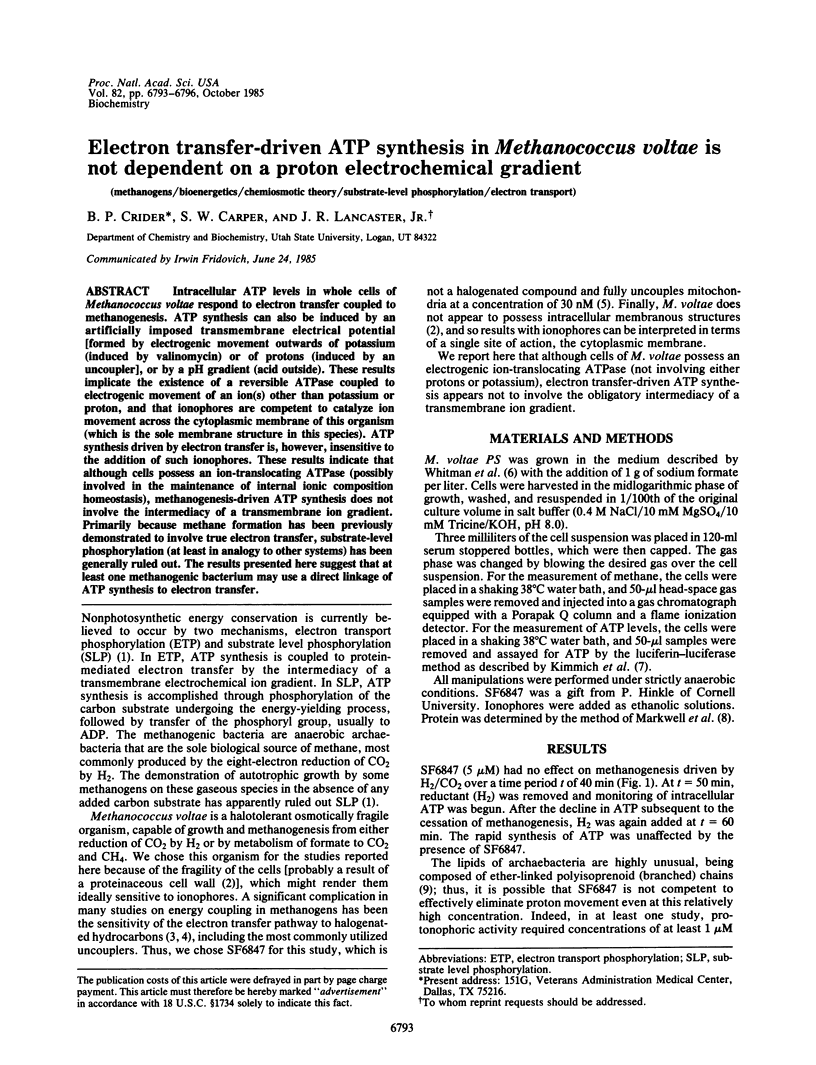
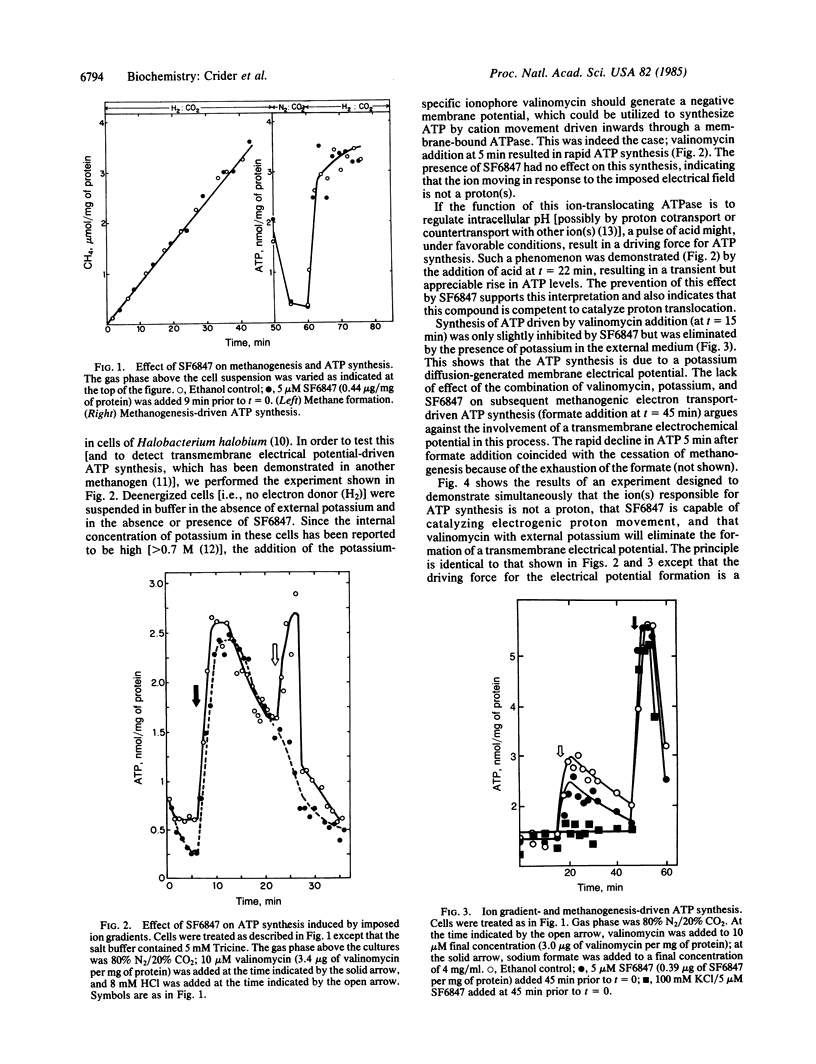
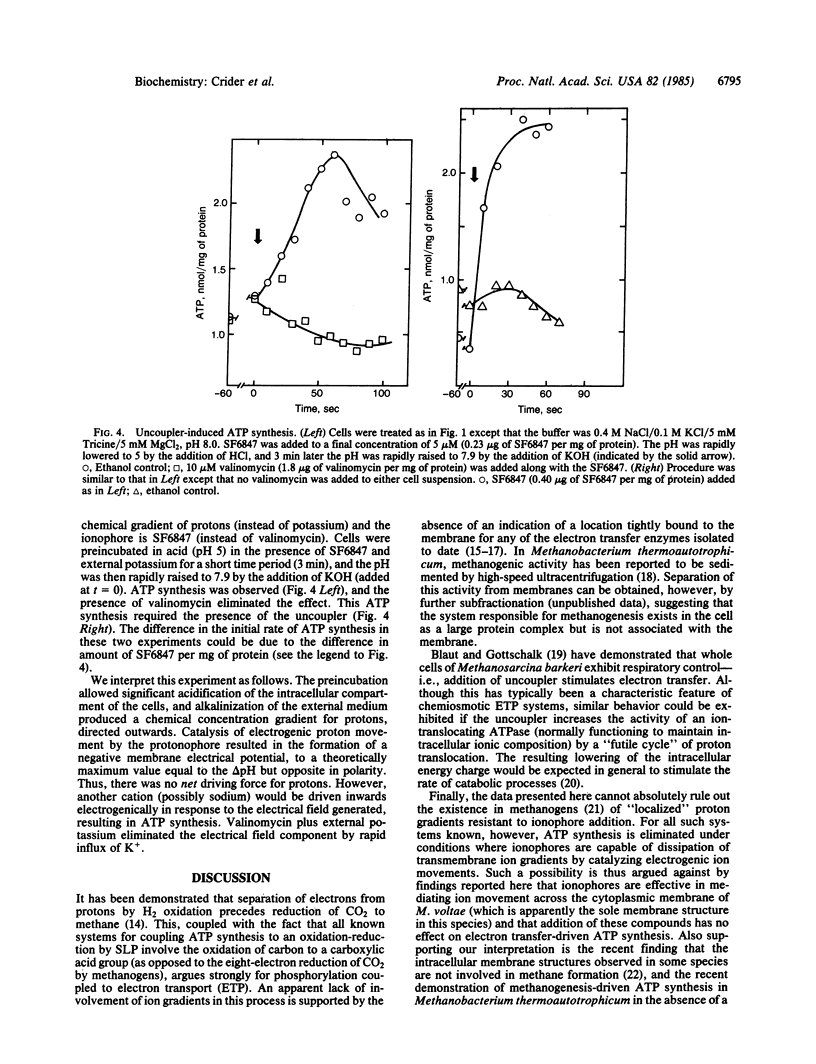
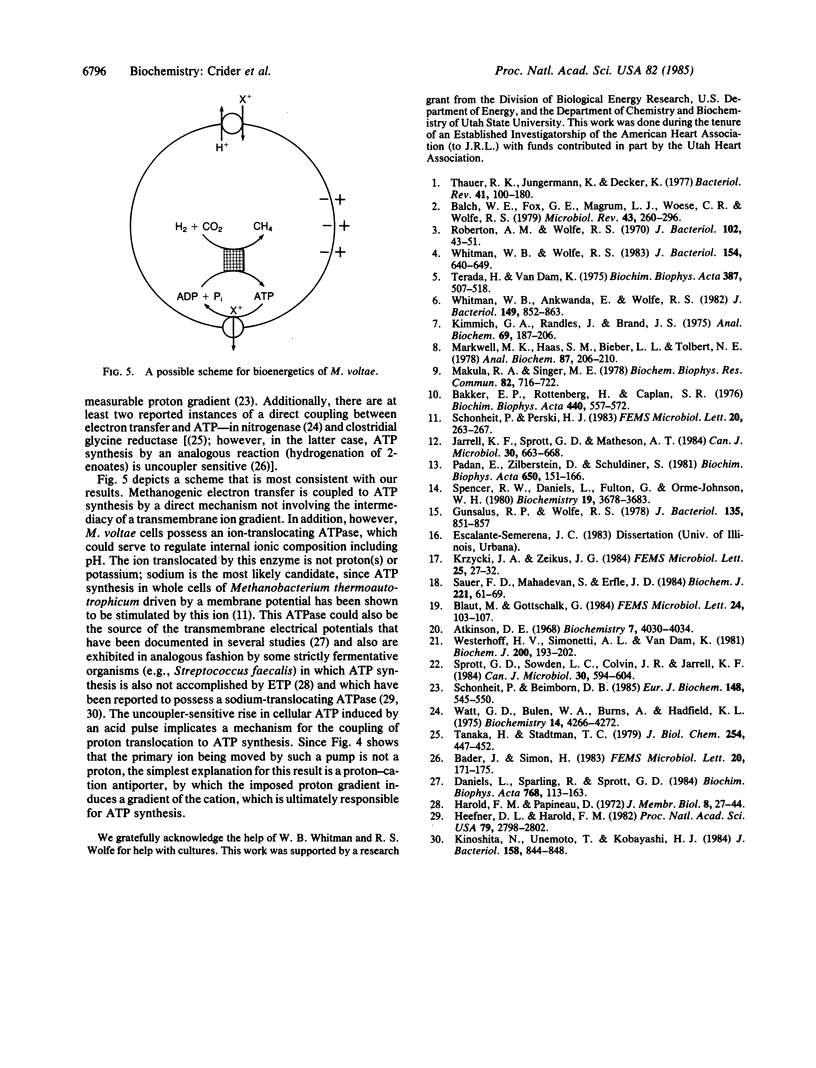
Selected References
These references are in PubMed. This may not be the complete list of references from this article.
- Atkinson D. E. The energy charge of the adenylate pool as a regulatory parameter. Interaction with feedback modifiers. Biochemistry. 1968 Nov;7(11):4030–4034. doi: 10.1021/bi00851a033. [DOI] [PubMed] [Google Scholar]
- Bakker E. P., Rottenberg H., Caplan S. R. An estimation of the light-induced electrochemical potential difference of protons across the membrane of Halobacterium halobium. Biochim Biophys Acta. 1976 Sep 13;440(3):557–572. doi: 10.1016/0005-2728(76)90042-6. [DOI] [PubMed] [Google Scholar]
- Balch W. E., Fox G. E., Magrum L. J., Woese C. R., Wolfe R. S. Methanogens: reevaluation of a unique biological group. Microbiol Rev. 1979 Jun;43(2):260–296. doi: 10.1128/mr.43.2.260-296.1979. [DOI] [PMC free article] [PubMed] [Google Scholar]
- Daniels L., Sparling R., Sprott G. D. The bioenergetics of methanogenesis. Biochim Biophys Acta. 1984 Sep 6;768(2):113–163. doi: 10.1016/0304-4173(84)90002-8. [DOI] [PubMed] [Google Scholar]
- Gunsalus R. P., Wolfe R. S. ATP activation and properties of the methyl coenzyme M reductase system in Methanobacterium thermoautotrophicum. J Bacteriol. 1978 Sep;135(3):851–857. doi: 10.1128/jb.135.3.851-857.1978. [DOI] [PMC free article] [PubMed] [Google Scholar]
- Harold F. M., Papineau D. Cation transport and electrogenesis by Streptococcus faecalis. I. The membrane potential. J Membr Biol. 1972;8(1):27–44. doi: 10.1007/BF01868093. [DOI] [PubMed] [Google Scholar]
- Heefner D. L., Harold F. M. ATP-driven sodium pump in Streptococcus faecalis. Proc Natl Acad Sci U S A. 1982 May;79(9):2798–2802. doi: 10.1073/pnas.79.9.2798. [DOI] [PMC free article] [PubMed] [Google Scholar]
- Kimmich G. A., Randles J., Brand J. S. Assay of picomole amounts of ATP, ADP, and AMP using the luciferase enzyme system. Anal Biochem. 1975 Nov;69(1):187–206. doi: 10.1016/0003-2697(75)90580-1. [DOI] [PubMed] [Google Scholar]
- Kinoshita N., Unemoto T., Kobayashi H. Sodium-stimulated ATPase in Streptococcus faecalis. J Bacteriol. 1984 Jun;158(3):844–848. doi: 10.1128/jb.158.3.844-848.1984. [DOI] [PMC free article] [PubMed] [Google Scholar]
- Makula R. A., Singer M. E. Ether-containing lipids of methanogenic bacteria. Biochem Biophys Res Commun. 1978 May 30;82(2):716–722. doi: 10.1016/0006-291x(78)90933-6. [DOI] [PubMed] [Google Scholar]
- Markwell M. A., Haas S. M., Bieber L. L., Tolbert N. E. A modification of the Lowry procedure to simplify protein determination in membrane and lipoprotein samples. Anal Biochem. 1978 Jun 15;87(1):206–210. doi: 10.1016/0003-2697(78)90586-9. [DOI] [PubMed] [Google Scholar]
- Padan E., Zilberstein D., Schuldiner S. pH homeostasis in bacteria. Biochim Biophys Acta. 1981 Dec;650(2-3):151–166. doi: 10.1016/0304-4157(81)90004-6. [DOI] [PubMed] [Google Scholar]
- Roberton A. M., Wolfe R. S. Adenosine triphosphate pools in Methanobacterium. J Bacteriol. 1970 Apr;102(1):43–51. doi: 10.1128/jb.102.1.43-51.1970. [DOI] [PMC free article] [PubMed] [Google Scholar]
- Sauer F. D., Mahadevan S., Erfle J. D. Methane synthesis by membrane vesicles and a cytoplasmic cofactor isolated from Methanobacterium thermoautotrophicum. Biochem J. 1984 Jul 1;221(1):61–69. doi: 10.1042/bj2210061. [DOI] [PMC free article] [PubMed] [Google Scholar]
- Schönheit P., Beimborn D. B. ATP synthesis in Methanobacterium thermoautotrophicum coupled to CH4 formation from H2 and CO2 in the apparent absence of an electrochemical proton potential across the cytoplasmic membrane. Eur J Biochem. 1985 May 2;148(3):545–550. doi: 10.1111/j.1432-1033.1985.tb08874.x. [DOI] [PubMed] [Google Scholar]
- Spencer R. W., Daniels L., Fulton G., Orme-Johnson W. H. Product isotope effects on in vivo methanogenesis by Methanobacterium thermoautotrophicum. Biochemistry. 1980 Aug 5;19(16):3678–3683. doi: 10.1021/bi00557a007. [DOI] [PubMed] [Google Scholar]
- Tanaka H., Stadtman T. C. Selenium-dependent clostridial glycine reductase. Purification and characterization of the two membrane-associated protein components. J Biol Chem. 1979 Jan 25;254(2):447–452. [PubMed] [Google Scholar]
- Terada H., VAN Dam K. On the stoichiometry between uncouplers of oxidative phosphorylation and respiratory chains. The catalytic action of SF 6847 (3,5-di-tert-butyl-4-hydroxy-benzylidenemalononitrile). Biochim Biophys Acta. 1975 Jun 17;387(3):507–518. doi: 10.1016/0005-2728(75)90089-4. [DOI] [PubMed] [Google Scholar]
- Thauer R. K., Jungermann K., Decker K. Energy conservation in chemotrophic anaerobic bacteria. Bacteriol Rev. 1977 Mar;41(1):100–180. doi: 10.1128/br.41.1.100-180.1977. [DOI] [PMC free article] [PubMed] [Google Scholar]
- Watt G. D., Bulen W. A., Burns A., Hadfield K. L. Stoichiometry, ATP/2e values, and energy requirements for reactions catalyzed by nitrogenase from Azotobacter vinelandii. Biochemistry. 1975 Sep 23;14(19):4266–4272. doi: 10.1021/bi00690a019. [DOI] [PubMed] [Google Scholar]
- Westerhoff H. V., Simonetti A. L., Van Dam K. The hypothesis of localized chemiosmosis is unsatisfactory. Biochem J. 1981 Nov 15;200(2):193–202. doi: 10.1042/bj2000193. [DOI] [PMC free article] [PubMed] [Google Scholar]
- Whitman W. B., Ankwanda E., Wolfe R. S. Nutrition and carbon metabolism of Methanococcus voltae. J Bacteriol. 1982 Mar;149(3):852–863. doi: 10.1128/jb.149.3.852-863.1982. [DOI] [PMC free article] [PubMed] [Google Scholar]
- Whitman W. B., Wolfe R. S. Activation of the methylreductase system from Methanobacterium bryantii by ATP. J Bacteriol. 1983 May;154(2):640–649. doi: 10.1128/jb.154.2.640-649.1983. [DOI] [PMC free article] [PubMed] [Google Scholar]