Abstract
The standard one-dimensional Rall cable model assumes that the electrotonic structure of neurons does not change in response to synaptic input. This model is used in a great number of both theoretical and anatomical-physiological structure-function studies. In particular, the membrane time constant, tau m, the somatic input resistance, Rin, and the electrotonic length are used to characterize single cells. However, these studies do not take into account that neurons are embedded in a network of spontaneously active cells. Synapses from these cells will contribute significantly to the membrane conductance, especially if recent evidence of very high specific membrane resistance, Rm = 100 k omega.cm2, is taken into account. We numerically simulated the electrical behavior of an anatomically reconstructed layer V cortical pyramidal cell receiving input from 4000 excitatory and 1000 inhibitory cells firing spontaneously at 0-7 Hz. We found that, over this range of synaptic background activity, tau m and Rin change by a factor of 10 (80-7 msec, 110-14 M omega) and the electrotonic length of the cell changes by a factor of 3. We show that this significantly changes the response of the cell to temporal desynchronized versus temporal synchronized synaptic input distributed throughout the neuron. Thus, the global activity of the network can control how individual cells perform spatial and temporal integration.
Full text
PDF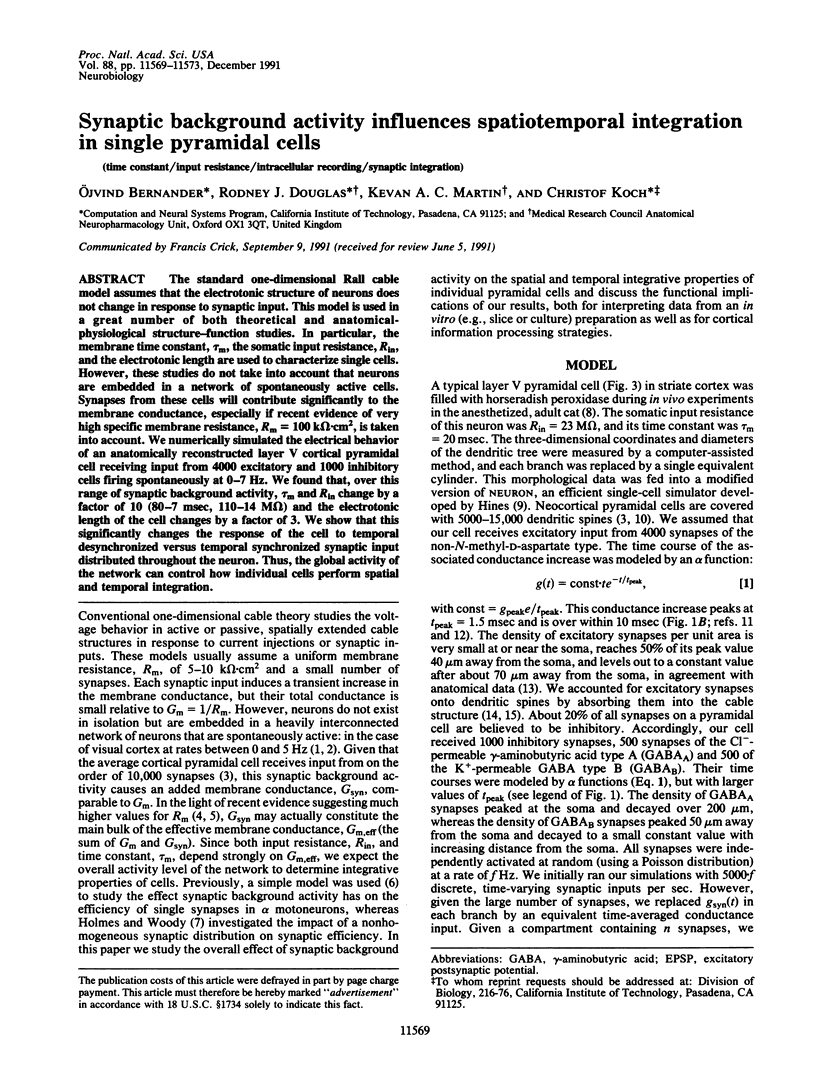
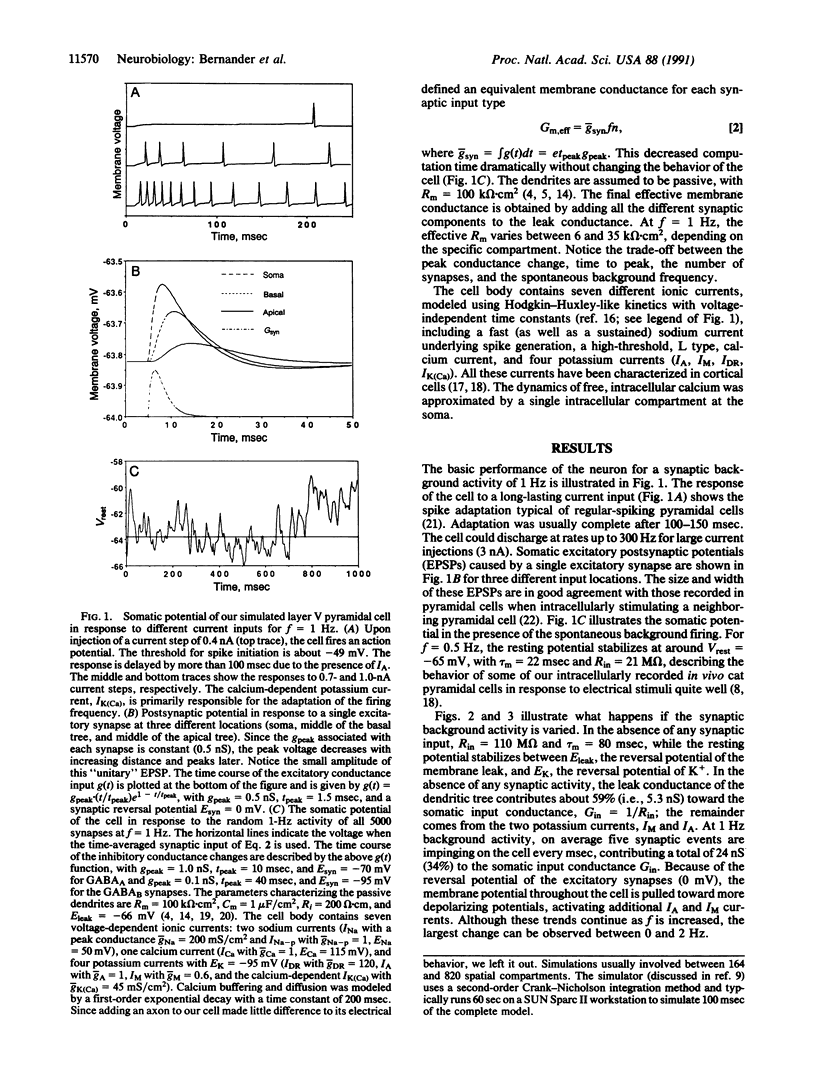
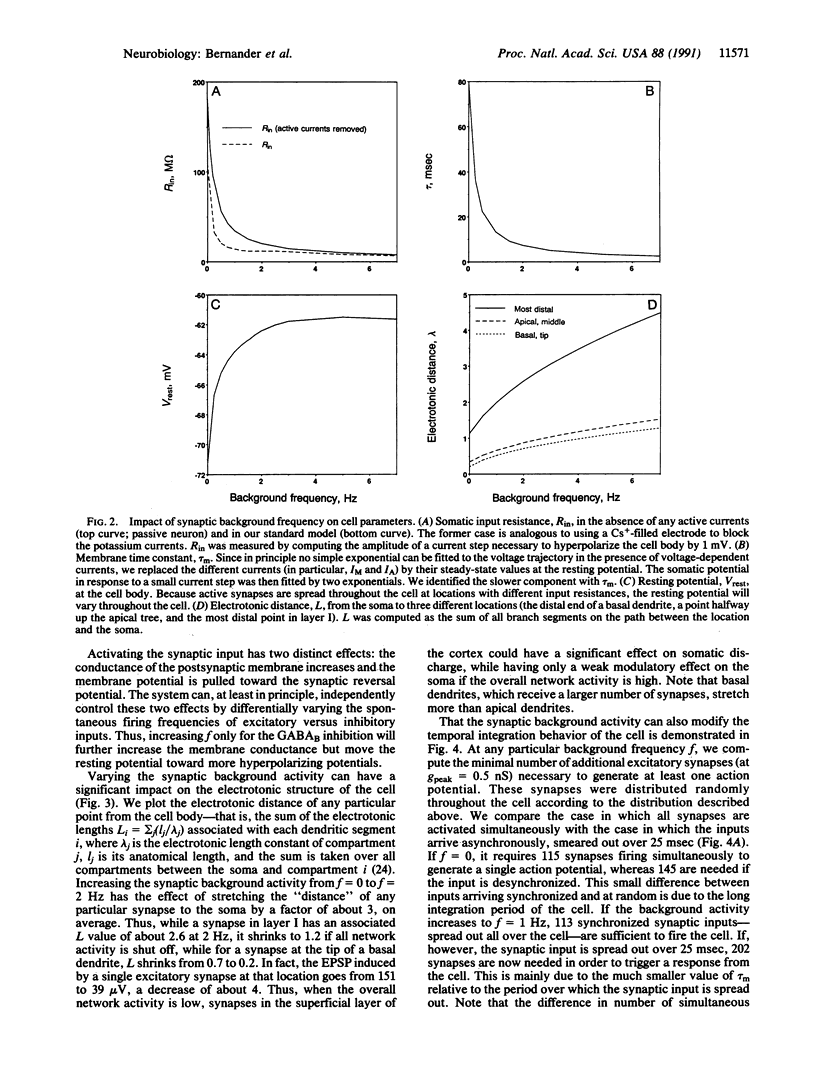
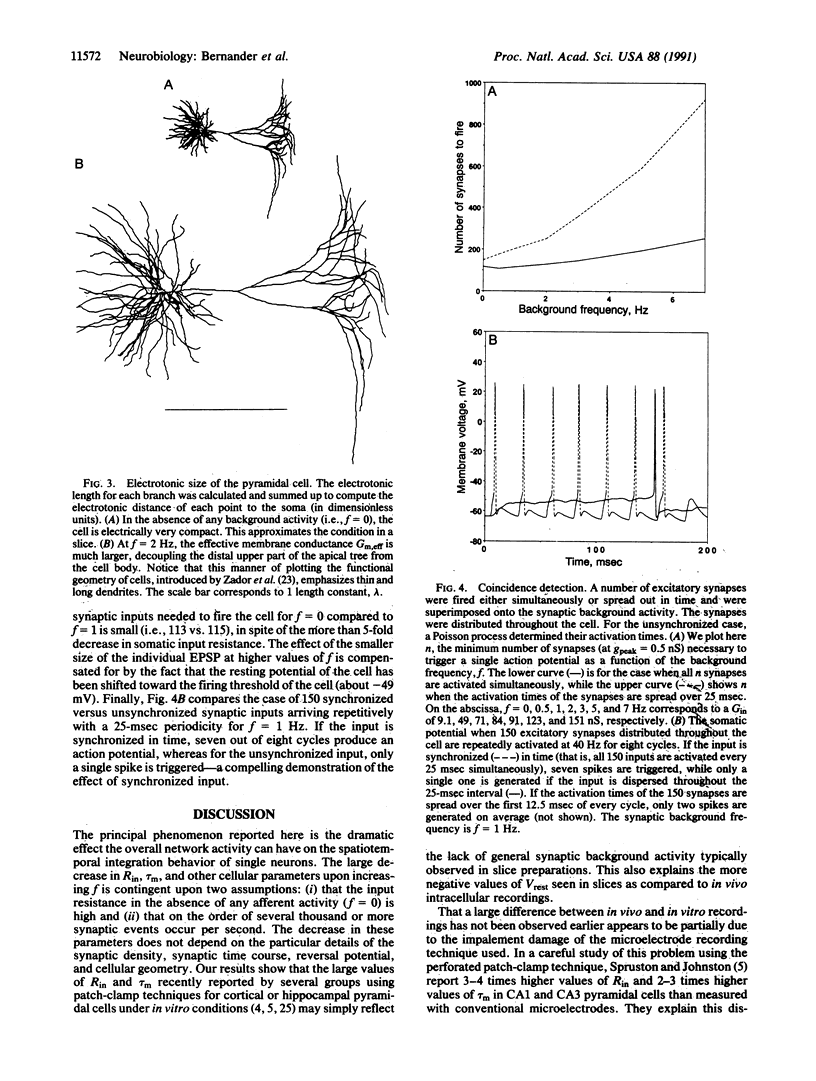
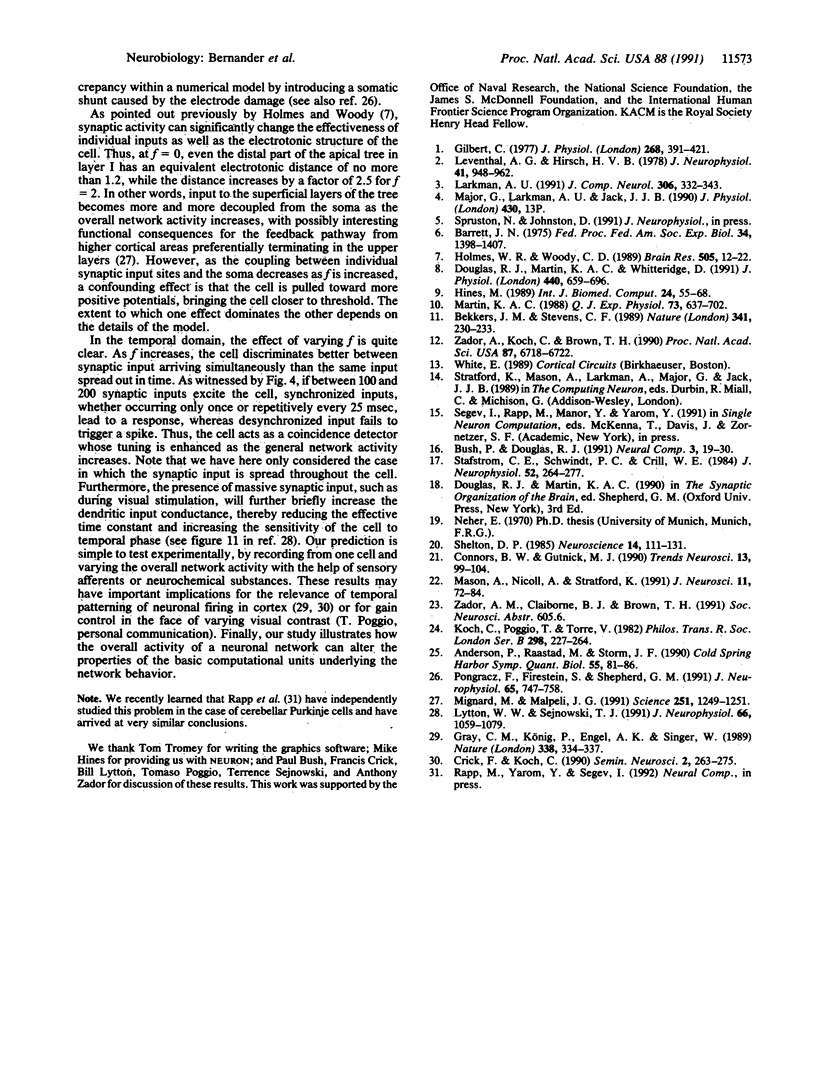
Selected References
These references are in PubMed. This may not be the complete list of references from this article.
- Andersen P., Raastad M., Storm J. F. Excitatory synaptic integration in hippocampal pyramids and dentate granule cells. Cold Spring Harb Symp Quant Biol. 1990;55:81–86. doi: 10.1101/sqb.1990.055.01.010. [DOI] [PubMed] [Google Scholar]
- Barrett J. N. Motoneuron dendrites: role in synaptic integration. Fed Proc. 1975 Apr;34(5):1398–1407. [PubMed] [Google Scholar]
- Bekkers J. M., Stevens C. F. NMDA and non-NMDA receptors are co-localized at individual excitatory synapses in cultured rat hippocampus. Nature. 1989 Sep 21;341(6239):230–233. doi: 10.1038/341230a0. [DOI] [PubMed] [Google Scholar]
- Connors B. W., Gutnick M. J. Intrinsic firing patterns of diverse neocortical neurons. Trends Neurosci. 1990 Mar;13(3):99–104. doi: 10.1016/0166-2236(90)90185-d. [DOI] [PubMed] [Google Scholar]
- Douglas R. J., Martin K. A., Whitteridge D. An intracellular analysis of the visual responses of neurones in cat visual cortex. J Physiol. 1991;440:659–696. doi: 10.1113/jphysiol.1991.sp018730. [DOI] [PMC free article] [PubMed] [Google Scholar]
- Gilbert C. D. Laminar differences in receptive field properties of cells in cat primary visual cortex. J Physiol. 1977 Jun;268(2):391–421. doi: 10.1113/jphysiol.1977.sp011863. [DOI] [PMC free article] [PubMed] [Google Scholar]
- Gray C. M., König P., Engel A. K., Singer W. Oscillatory responses in cat visual cortex exhibit inter-columnar synchronization which reflects global stimulus properties. Nature. 1989 Mar 23;338(6213):334–337. doi: 10.1038/338334a0. [DOI] [PubMed] [Google Scholar]
- Hines M. A program for simulation of nerve equations with branching geometries. Int J Biomed Comput. 1989 Mar;24(1):55–68. doi: 10.1016/0020-7101(89)90007-x. [DOI] [PubMed] [Google Scholar]
- Holmes W. R., Woody C. D. Effects of uniform and non-uniform synaptic 'activation-distributions' on the cable properties of modeled cortical pyramidal neurons. Brain Res. 1989 Dec 25;505(1):12–22. doi: 10.1016/0006-8993(89)90110-8. [DOI] [PubMed] [Google Scholar]
- Hurst A. M., Hunter M. Stretch-activated channels in single early distal tubule cells of the frog. J Physiol. 1990 Nov;430:13–24. doi: 10.1113/jphysiol.1990.sp018278. [DOI] [PMC free article] [PubMed] [Google Scholar]
- Koch C., Poggio T., Torre V. Retinal ganglion cells: a functional interpretation of dendritic morphology. Philos Trans R Soc Lond B Biol Sci. 1982 Jul 27;298(1090):227–263. doi: 10.1098/rstb.1982.0084. [DOI] [PubMed] [Google Scholar]
- Larkman A. U. Dendritic morphology of pyramidal neurones of the visual cortex of the rat: III. Spine distributions. J Comp Neurol. 1991 Apr 8;306(2):332–343. doi: 10.1002/cne.903060209. [DOI] [PubMed] [Google Scholar]
- Leventhal A. G., Hirsch H. V. Receptive-field properties of neurons in different laminae of visual cortex of the cat. J Neurophysiol. 1978 Jul;41(4):948–962. doi: 10.1152/jn.1978.41.4.948. [DOI] [PubMed] [Google Scholar]
- Lytton W. W., Sejnowski T. J. Simulations of cortical pyramidal neurons synchronized by inhibitory interneurons. J Neurophysiol. 1991 Sep;66(3):1059–1079. doi: 10.1152/jn.1991.66.3.1059. [DOI] [PubMed] [Google Scholar]
- Martin K. A. The Wellcome Prize lecture. From single cells to simple circuits in the cerebral cortex. Q J Exp Physiol. 1988 Sep;73(5):637–702. doi: 10.1113/expphysiol.1988.sp003190. [DOI] [PubMed] [Google Scholar]
- Mason A., Nicoll A., Stratford K. Synaptic transmission between individual pyramidal neurons of the rat visual cortex in vitro. J Neurosci. 1991 Jan;11(1):72–84. doi: 10.1523/JNEUROSCI.11-01-00072.1991. [DOI] [PMC free article] [PubMed] [Google Scholar]
- Mignard M., Malpeli J. G. Paths of information flow through visual cortex. Science. 1991 Mar 8;251(4998):1249–1251. doi: 10.1126/science.1848727. [DOI] [PubMed] [Google Scholar]
- Pongracz F., Firestein S., Shepherd G. M. Electrotonic structure of olfactory sensory neurons analyzed by intracellular and whole cell patch techniques. J Neurophysiol. 1991 Mar;65(3):747–758. doi: 10.1152/jn.1991.65.3.747. [DOI] [PubMed] [Google Scholar]
- Shelton D. P. Membrane resistivity estimated for the Purkinje neuron by means of a passive computer model. Neuroscience. 1985 Jan;14(1):111–131. doi: 10.1016/0306-4522(85)90168-x. [DOI] [PubMed] [Google Scholar]
- Stafstrom C. E., Schwindt P. C., Crill W. E. Repetitive firing in layer V neurons from cat neocortex in vitro. J Neurophysiol. 1984 Aug;52(2):264–277. doi: 10.1152/jn.1984.52.2.264. [DOI] [PubMed] [Google Scholar]
- Zador A., Koch C., Brown T. H. Biophysical model of a Hebbian synapse. Proc Natl Acad Sci U S A. 1990 Sep;87(17):6718–6722. doi: 10.1073/pnas.87.17.6718. [DOI] [PMC free article] [PubMed] [Google Scholar]