Abstract
The final lengths of intact dark-grown coleoptiles vary with species and cultivar. The growth distribution pattern in the apical 25-mm growing zone and the absolute amount of growth in each zone depend on the age and species of the coleoptile. A comparative study of several cultivars of wheat, Triticum vulgare, and barley, Hordeum vulgare, indicates that the growth distribution pattern in 30- to 38-mm coleoptiles varies with the species and cultivar. In barley, there are two patterns of growth distribution among the several cultivars, whereas in wheat, all cultivars exhibit a common zonal growth pattern. The total growth of coleoptiles, initially 30 to 38 mm in length, during a 24-hour dark incubation period is the same in dark-grown coleoptiles as in those irradiated with 3 minutes of red (660 nm) light prior to the incubation period. The growth distribution pattern in the growing zone of this 30- to 38-mm coleoptile is, however, altered by red light. Growth of the apical 5-mm zone is stimulated by red light and the zonal growth 5 to 10 mm below the apex is only slightly affected, whereas growth in the zones 10 to 15 to 20, and 20 to 25 mm below the apex is inhibited. This growth distribution pattern in irradiated coleoptiles changes as the coleoptile increases in length. The response of a zone following exposure to red light is dependent upon the age of the seedlings irradiated. The over-all effect of red light on growth of the intact coleoptile varies with the length of the coleoptile. In young seedling 20 to 29 mm in length, the cells of the coleoptile can compensate for the effects of red light, with the over-all growth of the dark-grown and irradiated coleoptile about the same. As the seedling grows older, the cells of the coleoptile can no longer make up for the effects of red light, and the over-all effect changes from compensation to pronounced inhibition.
Full text
PDF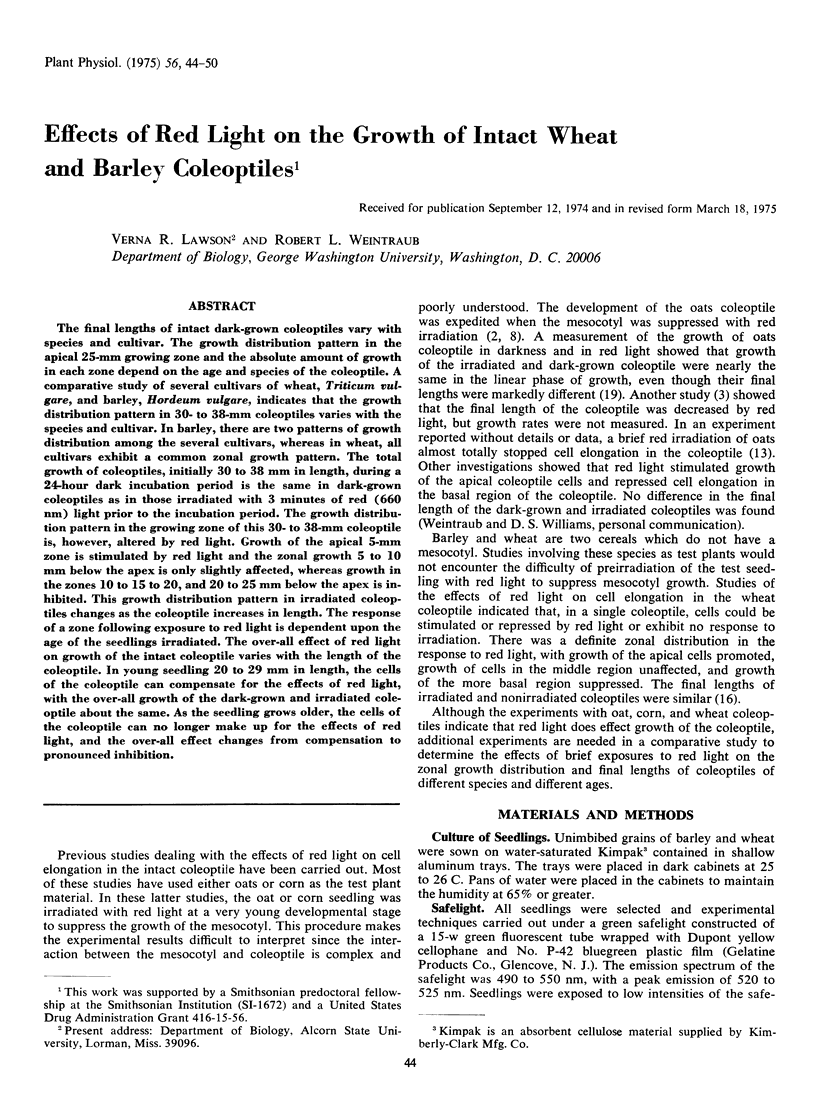
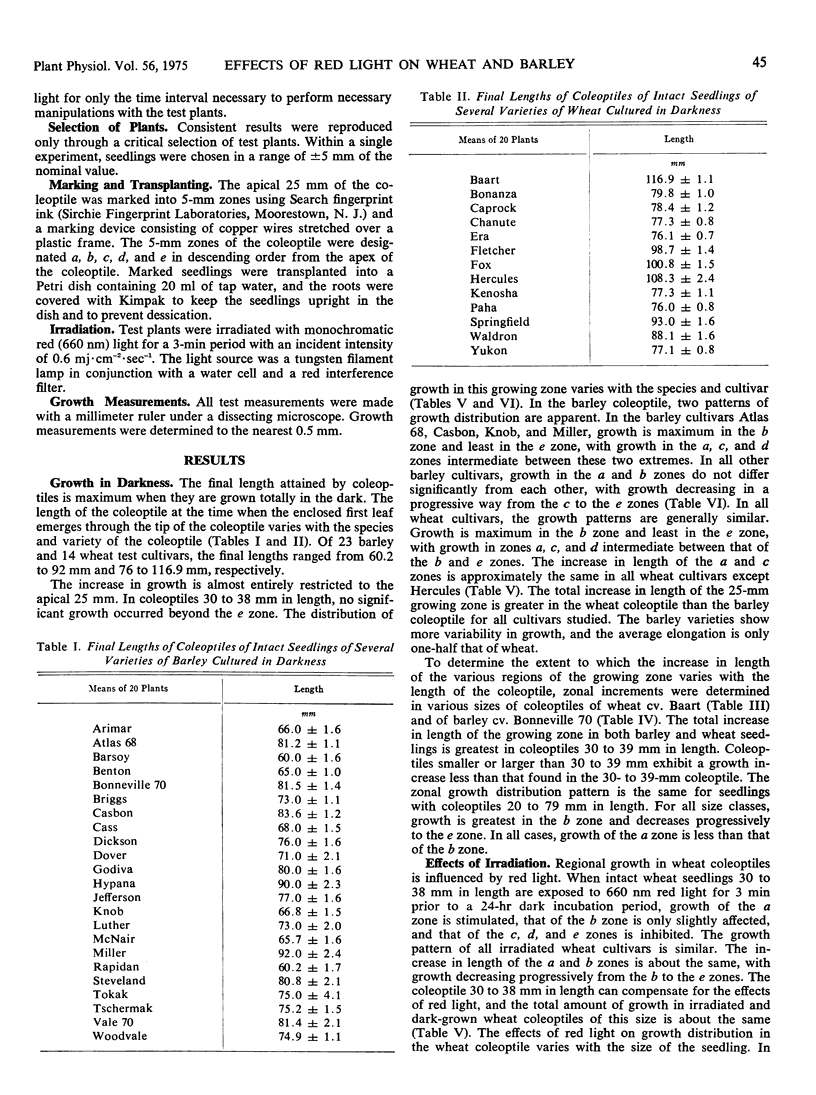
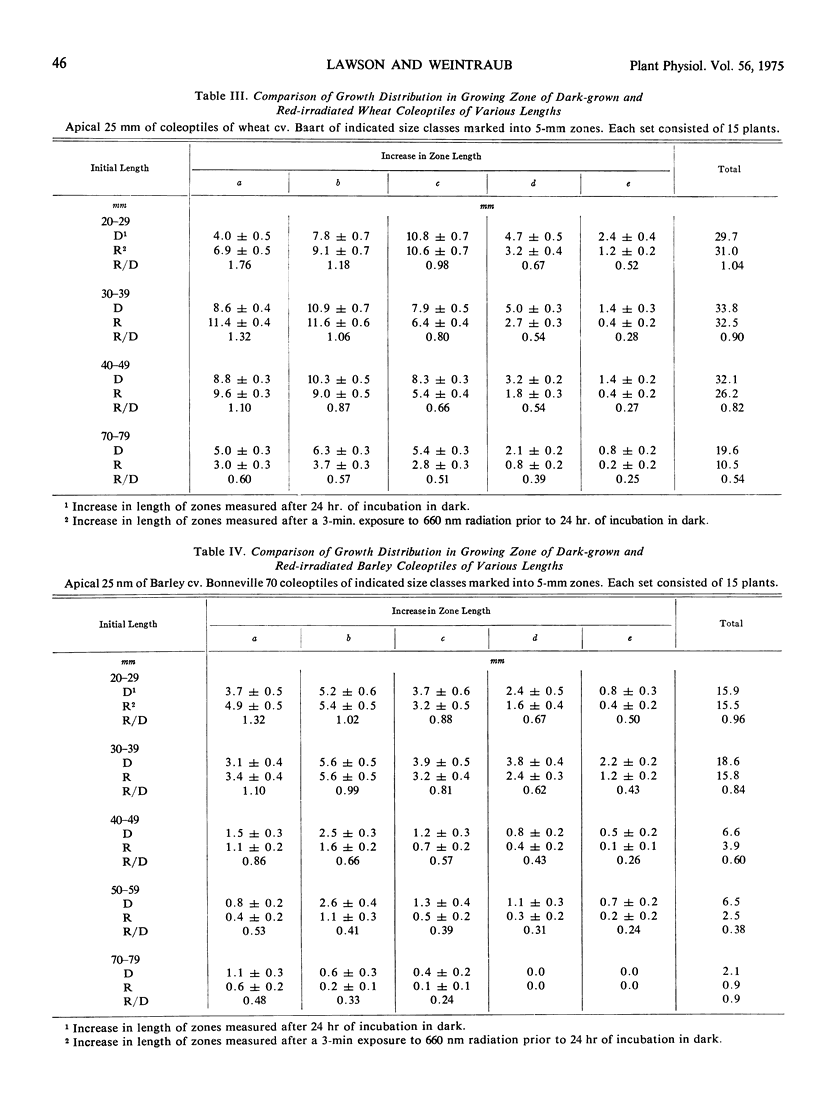
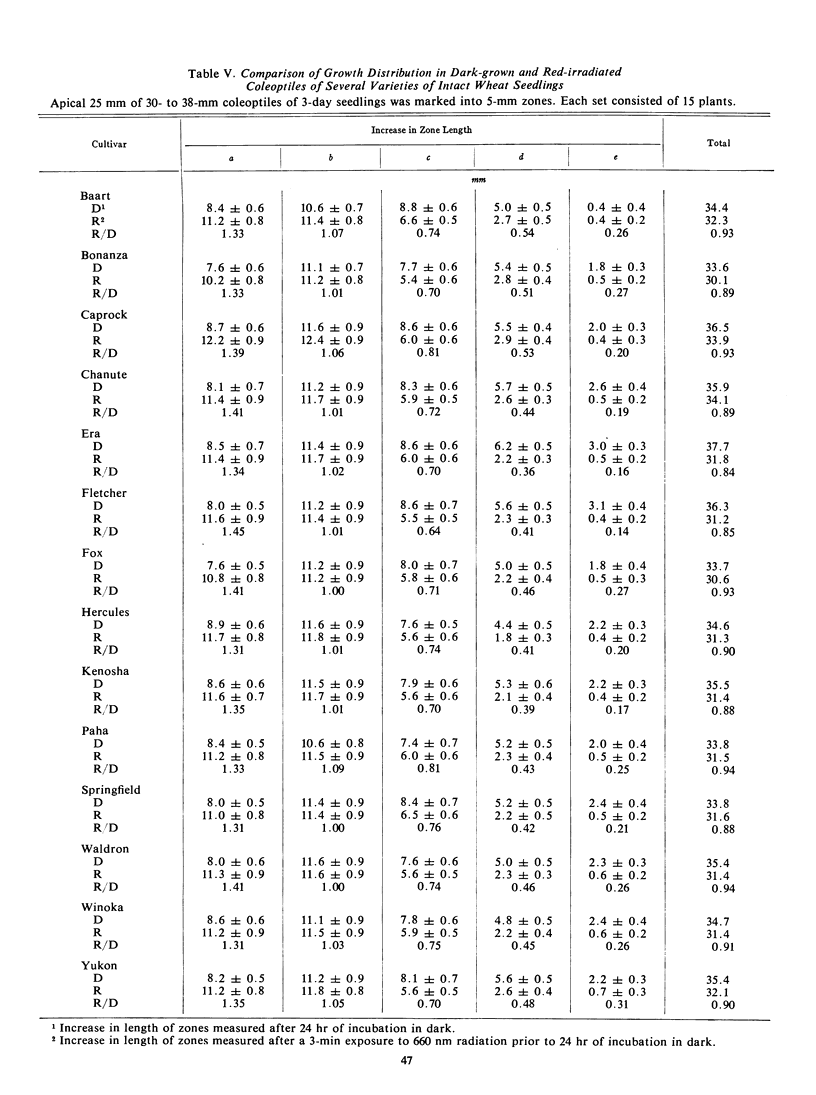
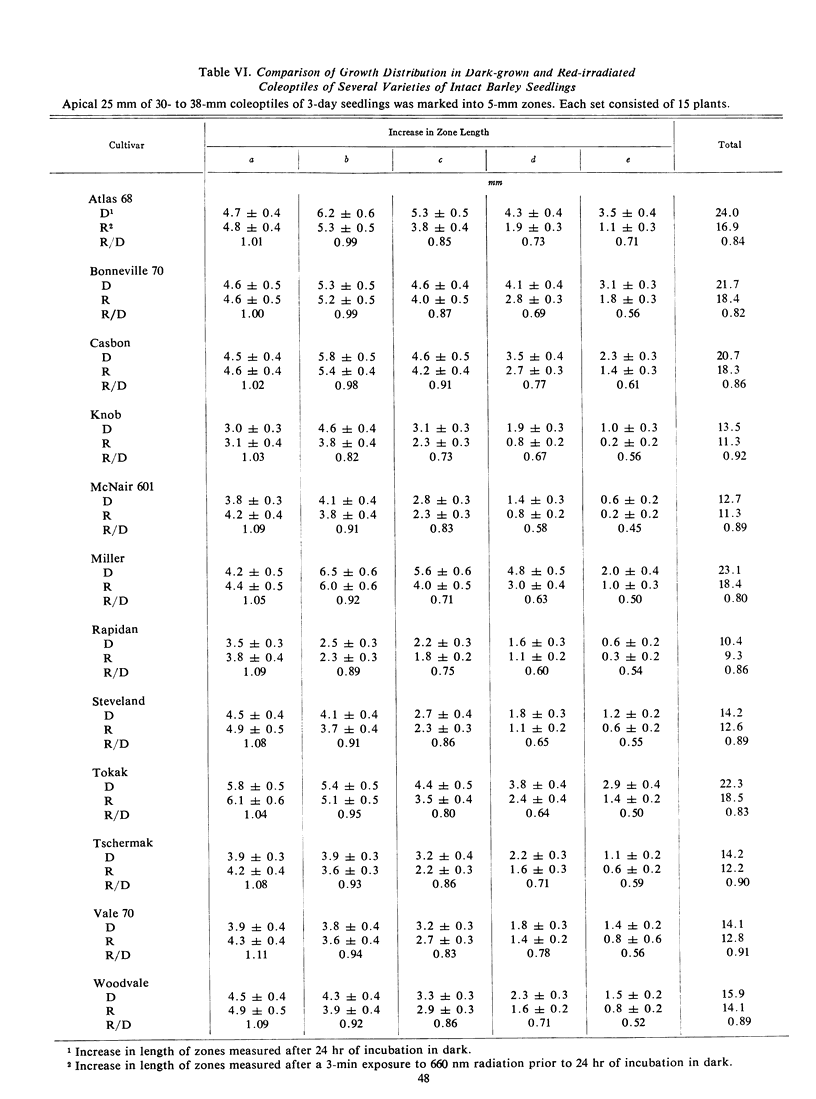
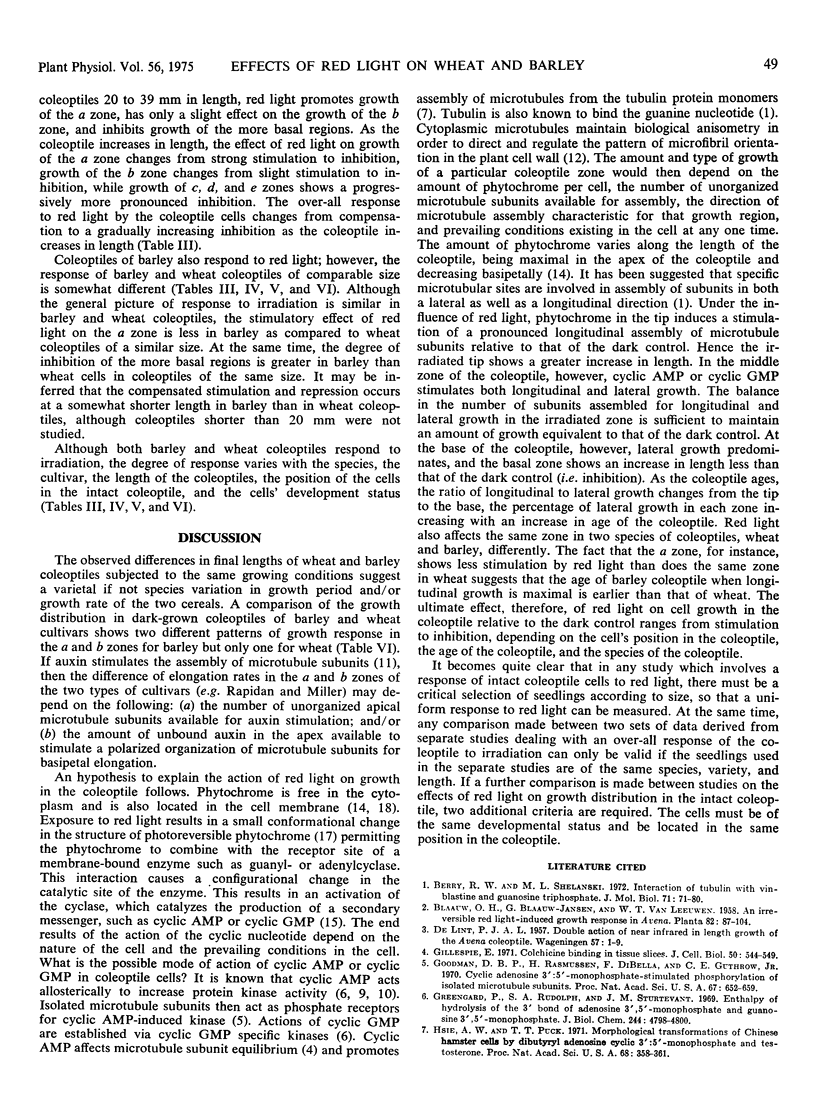
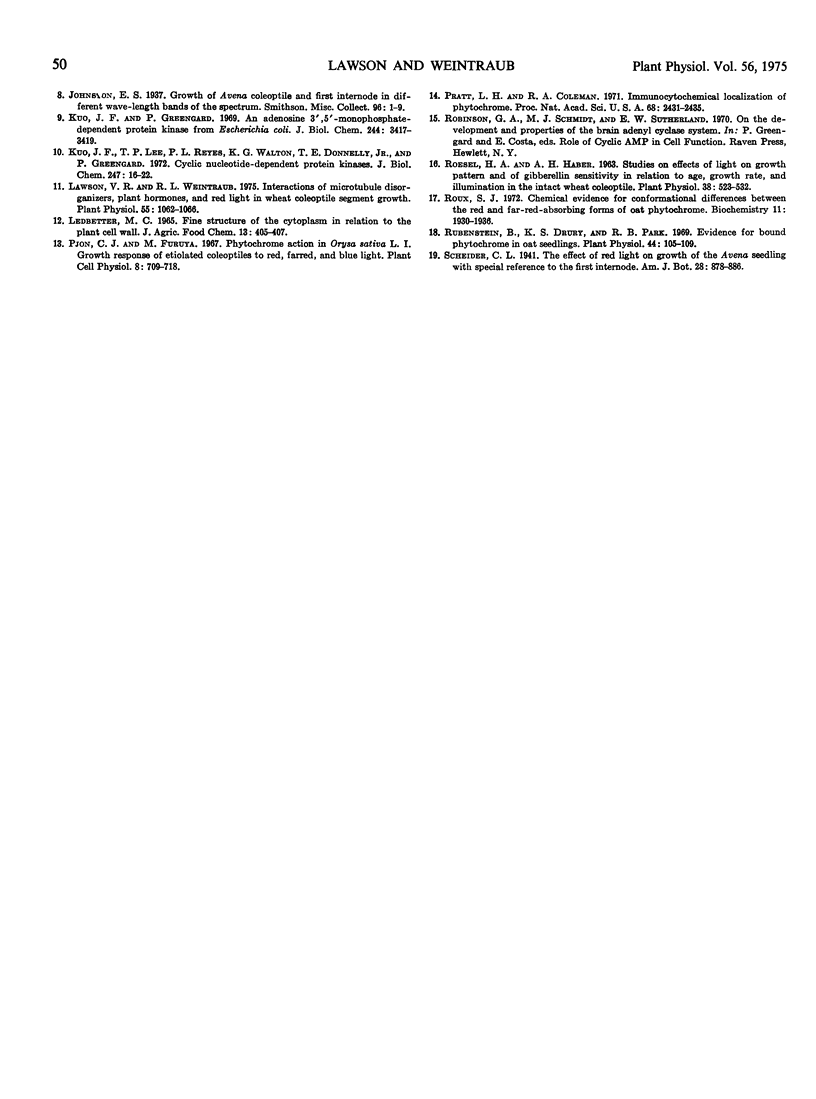
Selected References
These references are in PubMed. This may not be the complete list of references from this article.
- Berry R. W., Shelanski M. L. Interactions of tubulin with vinblastine and guanosine triphosphate. J Mol Biol. 1972 Oct 28;71(1):71–80. doi: 10.1016/0022-2836(72)90401-9. [DOI] [PubMed] [Google Scholar]
- Gillespie E. Colchicine binding in tissue slices. Decrease by calcium and biphasic effect of adenosine-3', 5'-monophosphate. J Cell Biol. 1971 Aug;50(2):544–549. doi: 10.1083/jcb.50.2.544. [DOI] [PMC free article] [PubMed] [Google Scholar]
- Goodman D. B., Rasmussen H., DiBella F., Guthrow C. E., Jr Cyclic adenosine 3':5'-monophosphate-stimulated phosphorylation of isolated neurotubule subunits. Proc Natl Acad Sci U S A. 1970 Oct;67(2):652–659. doi: 10.1073/pnas.67.2.652. [DOI] [PMC free article] [PubMed] [Google Scholar]
- Greengard P., Rudolph S. A., Sturtevant J. M. Enthalpy of hydrolysis of the 3' bond of adenosine 3',5'-monophosphate and guanosine 3',5'-monophosphate. J Biol Chem. 1969 Sep 10;244(17):4798–4800. [PubMed] [Google Scholar]
- Hsie A. W., Puck T. T. Morphological transformation of Chinese hamster cells by dibutyryl adenosine cyclic 3':5'-monophosphate and testosterone. Proc Natl Acad Sci U S A. 1971 Feb;68(2):358–361. doi: 10.1073/pnas.68.2.358. [DOI] [PMC free article] [PubMed] [Google Scholar]
- Kuo J. F., Greengard P. An adenosine 3',5'-monophosphate-dependent protein kinase from Escherichia coli. J Biol Chem. 1969 Jun 25;244(12):3417–3419. [PubMed] [Google Scholar]
- Kuo J. F., Lee T. P., Reyes P. L., Walton K. G., Donnelly T. E., Jr, Greengard P. Cyclic nucleotide-dependent protein kinases. X. An assay method for the measurement of quanosine 3',5'-monophosphate in various biological materials and a study of agents regulating its levels in heart and brain. J Biol Chem. 1972 Jan 10;247(1):16–22. [PubMed] [Google Scholar]
- Lawson V. R., Weintraub R. L. Interactions of microtubule disorganizers, plant hormones, and red light in wheat coleoptile segment growth. Plant Physiol. 1975 Jun;55(6):1062–1066. doi: 10.1104/pp.55.6.1062. [DOI] [PMC free article] [PubMed] [Google Scholar]
- Pratt L. H., Coleman R. A. Immunocytochemical localization of phytochrome. Proc Natl Acad Sci U S A. 1971 Oct;68(10):2431–2435. doi: 10.1073/pnas.68.10.2431. [DOI] [PMC free article] [PubMed] [Google Scholar]
- Roesel H. A., Haber A. H. Studies of Effects of Light on Growth Pattern and of Gibberellin Sensitivity in Relation to Age, Growth Rate, and Illumination in Intact Wheat Coleoptiles. Plant Physiol. 1963 Sep;38(5):523–532. doi: 10.1104/pp.38.5.523. [DOI] [PMC free article] [PubMed] [Google Scholar]
- Roux S. J. Chemical evidence for conformational differences between the red- and far-red-absorbing forms of oat phytochrome. Biochemistry. 1972 May 9;11(10):1930–1936. doi: 10.1021/bi00760a030. [DOI] [PubMed] [Google Scholar]
- Rubinstein B., Drury K. S., Park R. B. Evidence for bound phytochrome in oat seedlings. Plant Physiol. 1969 Jan;44(1):105–109. doi: 10.1104/pp.44.1.105. [DOI] [PMC free article] [PubMed] [Google Scholar]